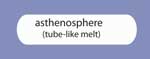 |
On the Origin of the Asthenosphere |
Shun-ichiro Karato
Department of Geology and Geophysics, Yale Universit, Kline Geology Lab., 210 Whitney Avenue, New Haven, CT 06520, USA,shun-ichiro.karato@yale.edu
This webpage is a summary of: Karato, Shun-ichiro, On the origin of the asthenosphere, Earth and Planetary Science Letters, 321–322, 95-103, 2012.
The asthenosphere is a “soft” layer beneath the hard lithosphere, and, as the name suggests, it is weak. In addition, it has unique geochemical characteristics: it is modestly “depleted” but nearly homogeneous in composition–it has a smaller amount of incompatible elements such as hydrogen compared to the source regions of ocean-island basalts (OIB).
There have been two paradigms to explain these features of the asthenosphere:
- the mechanical weakness is a result of the presence of a small amount of melt (e.g., Anderson & Spetzler, 1970), and;
- it is modestly depleted and nearly homogeneous in composition as a result of the mixing of highly depleted materials, following continental crust formation, with un-processed (undepleted) materials (Hofmann, 1988).
In this webpage, I review various models for the origin of the asthenosphere based on the latest observations of mineral physics, melting relationships, and thermal models of the upper mantle. I conclude that partial melting probably occurs throughout the upper mantle, except for in the lithosphere. However, in order to explain the inferred shallow lithosphere-asthenosphere boundary (LAB) with its large and sharp velocity reduction in old oceanic mantle by partial melting (e.g., Kawakatsu et al., 2009; Rychert & Shearer, 2009), a high-end geotherm and mechanisms for melt accumulation are required. A purely thermal sub-solidus model of the asthenosphere (e.g., Faul & Jackson, 2005; Schubert et al., 1976; Stixrude & Lithgow-Bertelloni, 2005) is inconsistent with the sharp LAB. A sub-solidus model invoking hydrogen stratification (Karato & Jung, 1998) can explain the shallow and sharp LAB, but it fails to explain the large velocity drop if the absorption-band model of anelasticity is assumed.
A review of recent literature (e.g., (Ghahremani, 1980; Morris & Jackson, 2009; Raj, 1975) shows that grain-size sensitive anelastic relaxation inevitably involves two successive processes: high-frequency elastically accommodated grain-boundary sliding, followed by low-frequency diffusion-related anelasticity. Anelastic relaxation caused by grain-boundary sliding leads to a large velocity reduction (~5-10%). Assuming a plausible temperature and water content dependence of the peak frequency of this relaxation, a substantial velocity reduction occurs at a nearly constant depth (~70 km) in old oceanic regions but at the age-dependent depth corresponding to the ~1300 K isotherm in the young oceans (Figure 1). This model also provides an explanation for the seismological observations on the cratonic upper mantle (Abt et al., 2010; Fischer et al., 2010) including the observed large velocity drop at the mid-lithosphere discontinuity and a small velocity change at the LAB.
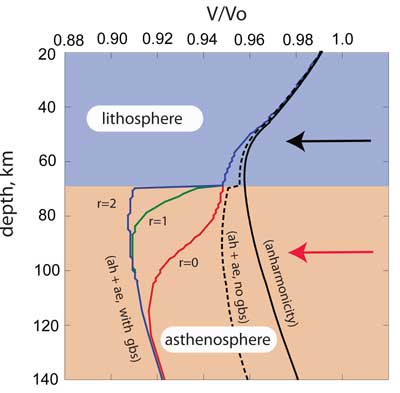
Figure 1: Seismic velocity vs. depth profiles in the upper mantle (100 Ma oceanic geotherm, half-space cooling model assumed). Black curve (anharmonicity): a model with only the effect of anharmonicity; broken blue curve: an absorption band model by Karato & Jung ( 1998); solid black curve: a model with grain-boundary sliding (r=2); solid green curve: a model with grain-boundary sliding (r=1); solid red curve: a model with grain-boundary sliding (r=0, no water effect). The sharp and large velocity drop at ~70 km can be explained when the influence of grain-boundary sliding is included with some sensitivity to water (r=1-2). In addition to the depth variation in velocity, substantial anisotropy exists in the upper mantle. Only azimuthal anisotropy is shown. Black arrow: the direction of plate motion; red arrow: the fast direction in this model. Radial anisotropy predicted by this model is (VSH-VSV)/<VS> ~ 2-5%; Karato et al., 2008).
A small amount of hydrogen (water) in the asthenosphere is explained, in this model, to result from a small degree of partial melting at ~410-km (Karato et al., 2006). I suggest that the upper mantle is made of the residual materials left after the small degree of partial melting at ~410 km depth is removed. Based on plausible models of partial melting, it is argued in (Karato et al., 2006) that only ~0.05 wt% of water is needed to cause partial melting at ~410 km depth. Recent work shows that the transition zone probably contains ~0.1-0.3 wt% of water (Karato, 2011). Consequently, partial melting is likely to occur at ~410 km depth. The inferred presence of a low-velocity region above 410 km (Tauzin et al., 2010) provides evidence for partial melting there.
The classic model of the composition of the asthenosphere (Hofmann, 1988) assumes nearly complete mixing of highly depleted and undepleted materials. Recent laboratory studies showed that depleted and undepleted materials have greatly differing viscosities because of the large difference in water content (e.g., (Karato & Jung, 2003), and thus complete mixing is unlikely (e.g., Manga, 1996). In my new model, the composition of the asthenosphere is explained as a result of partial melting at ~410 km. In this model, the composition of the residual materials is controlled by the melting relationship and is nearly homogenous even if the original materials have wide compositional variety. Since the degree of partial melting at 410 km depth is small (because the degree of melting is limited by the amount of water and other volatiles), it also explains the modestly depleted nature of the asthenosphere. The melt produced at ~410 km is initially dense, because of the high pressure (Matsukage et al., 2005), but becomes buoyant at a shallower depth. Above this depth, the melt will migrate to the bottom of the lithosphere and contribute to the mechanical decoupling of the lithosphere and the mantle below that seems to be required to explain trench parallel seismic anisotropy below the subducted slabs (Long & Silver, 2009).
In summary, I propose that most geophysical and geochemical observations concerning the asthenosphere can be explained by invoking a small degree of partial melting at ~410 km (Figure 2). A small degree of partial melting reduces the water content, but the asthenosphere still retains enough water to change its mechanical properties. The sharp and shallow LAB can be attributed partly to the influence of a small amount of water. Also the near-homogenous composition of the asthenosphere is attributed, in this model, to the “cleaning” (filtering) effect of partial melting.
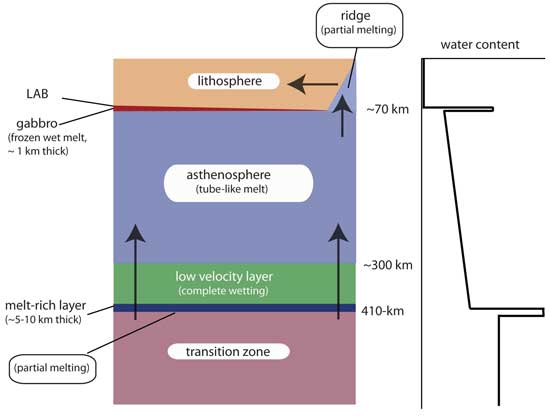
Figure 2: Schematic diagram showing the structure and processes affecting the asthenosphere. The water-content depth profile is based on Karato ( 2011) and is schematic). A low degree of partial melting occurs at ~ 410 km as a result of the high water content in the transition zone. The asthenosphere is the residual of this partial melting and contains ~0.01 wt% (depending on the depth) of water, which is enough to make it soft. Beneath the ridge, extensive partial melting occurs at ~70 km (Hirth & Kohlstedt, 1996) that forms highly depleted lithosphere defining the lithosphere-asthenosphere boundary at ~70 km. In the deep upper mantle (~300 km or deeper), a small amount of melt completely wets olivine grain-boundaries (Yoshino et al., 2007) causing a thick low-velocity region above 410 km (Tauzin et al., 2010). Near the 410-km discontinuity, the melt is probably denser than the surrounding minerals (Karato et al., 2006), and stays at 410 km or in the deep mantle (Bercovici & Karato, 2003). However, melt becomes buoyant at a shallower depth, and will rise to the bottom of the lithosphere, providing an explanation for “petit spots” (Hirano et al., 2007) and the lubrication needed to explain trench parallel anisotropy (Long & Silver, 2009).
References
-
Abt, D. L., K. M. Fischer, S. W. French, H. A. Ford, H. Yuan, and B. Romanowicz (2010), North American lithosphere discontinuity structure imaged by Ps and Sp receiver functions, Journal of Geophysical Research, 115, 10.1029/2009JB00691.
-
Anderson, D. L., and H. Spetzler (1970), Partial melting and the low-velocity zone, Physics of the Earth and Planetary Interiors, 4, 62-64.
-
Bercovici, D., and S. Karato (2003), Whole mantle convection and transition-zone water filter, Nature, 425, 39-44.
-
Faul, U. H., and I. Jackson (2005), The seismological signature of temperature and grain size variations in the upper mantle, Earth and Planetary Science Letters, 234, 119-134.
-
Fischer, K. M., H. A. Ford, D. L. Abt, and C. A. Rychert (2010), The lithosphere-asthenosphere boundary, Annual Review of Earth and Planetary Sciences, 38, 551-575.
-
Ghahremani, F. (1980), Effect of grain boundary sliding on anelasticity of polycrystals, International Journal of Solid Structures, 16, 825-845.
-
Hirano, N., et al. (2007), Volcanism in response to plate flexure, Science, 313, 1426-1428.
-
Hirth, G., and D. L. Kohlstedt (1996), Water in the oceanic upper mantle - implications for rheology, melt extraction and the evolution of the lithosphere, Earth and Planetary Science Letters, 144, 93-108.
-
Hofmann, A. W. (1988), Chemical differentiation of the Earth: the relationship between mantle, continental crust, and oceanic crust, Earth and Planetary Science Letters, 90, 297-314.
-
Karato, S. (2011), Water distribution across the mantle transition zone and its implications for global material circulation, Earth and Planetary Science Letters, 301, 413-423.
-
Karato, S., D. Bercovici, G. Leahy, G. Richard, and Z. Jing (2006), Transition zone water filter model for global material circulation: Where do we stand?, in Earth's Deep Water Cycle, edited by S. D. Jacobsen and S. van der Lee, pp. 289-313, American Geophysical Union, Washington DC.
-
Karato, S., and H. Jung (1998), Water, partial melting and the origin of seismic low velocity and high attenuation zone in the upper mantle, Earth and Planetary Science Letters, 157, 193-207.
-
Karato, S., and H. Jung (2003), Effects of pressure on high-temperature dislocation creep in olivine polycrystals, Philosophical Magazine, A., 83, 401-414.
-
Karato, S., H. Jung, I. Katayama, and P. A. Skemer (2008), Geodynamic significance of seismic anisotropy of the upper mantle: New insights from laboratory studies, Annual Review of Earth and Planetary Sciences, 36, 59-95.
-
Kawakatsu, H., P. Kumar, Y. Takei, M. Shinohara, T. Kanazawa, E. Araki, and K. Suyehiro (2009), Seismic evidence for sharp lithosphere-asthenosphere boundaries of oceanic plates, Science, 324, 499-502.
-
Long, M. D., and P. G. Silver (2009), Mantle flow in subduction systems: The subslab flow field and implications for mantle dynamics, Journal of Geophysical Research, 114, 10.1029/2008JB006200.
-
Manga, M. (1996), Mixing of heterogeneities in the mantle: effect of viscosity differences, Geophysical Research Letters, 23, 403-406.
-
Matsukage, K. N., Z. Jing, and S. Karato (2005), Density of hydrous silicate melt at the conditions of the Earth's deep upper mantle, Nature, 438, 488-491.
-
Morris, S. J. S., and I. Jackson (2009), Diffusionally assisted grain-boundary sliding and viscosity of polycrystals, Journal of the Mechanics and Physics of Solids, 57, 744-761.
-
Raj, R. (1975), Transient behavior of diffusion-induced creep and creep rupture, Metallurgical Transactions, 6A, 1499-1509.
-
Rychert, C. A., and P. M. Shearer (2009), A global view of the lithosphere-asthenosphere boundary, Science, 324, 495-498.
-
Schubert, G., C. Froidevaux, and D. A. Yuen (1976), Oceanic lithosphere: thermal and mechanical structure, Journal of Geophysical Research, 81, 3525-3540.
-
Stixrude, L., and C. Lithgow-Bertelloni (2005), Mineralogy and elasticity of the oceanic upper mantle: Origin of the low-velocity zone, Journal of Geophysical Research, 110, 10.1029/2004JB002965.
-
Tauzin, B., E. Debayle, and G. Wittingger (2010), Seismic evidence for a global low-velocity layer within the Earth's upper mantle, Nature Geoscience, 3, 718-721.
-
Yoshino, T., Y. Nishihara, and S. Karato (2007), Complete wetting of olivine grain-boundaries by a hydrous melt near the mantle transition zone Earth and Planetary Science Letters, 256, 466-472.
last updated 3rd March, 2012 |