 |
Boundary
layer fabric, dynamics and the origin
of midplate volcanoes |
|
|
Don L. Anderson
Seismological
Laboratory, California Institute of Technology, Pasadena,
CA 91125, dla@gps.caltech.edu
This webpage is an overview of the
LLAMA model (Anderson,
D.L., Hawaii, boundary layers and ambient mantle–geophysical
constraints, J.
Petrology, published online December 2, 2010,
doi: 10.1093/petrology/egq068, available on-line at
Journal of Petrology, Advanced Access).
The reference list
in that paper is updated at the end of this contribution
to include 2010 and 2011 publications.
LLAMA
The anisotropic seismic low-velocity
layer (LVL) in the mantle under ocean basins is delineated
by the Gutenberg (G) and Lehmann (L) discontinuities
at average depths of about 70 and 220 km depth, respectively.
A thinner, deeper, and less anisotropic LVL terminates
near 220 km under cratons. The 220 ± 20 km depth
level (L) is a universal and fundamental tectonic boundary
in the mantle. The mantle above this level, Gutenberg’s
Region B (Figure 1), is anisotropic because it is advected
and sheared by plate motions and contains aligned melt
arrays (Figure 2). Although it is weak and deforming,
it supports a conduction gradient and is therefore
part of the thermal boundary layer (TBL) and not
part of the so-called convecting mantle. The mantle
below this level is decoupled from plate motions and
provides a relatively fixed shallow reference system.
Oceanic volcanism taps magmas in the LVL, which ascend
by hydraulic magma fracturing rather than by thermal
erosion. Region B contains the key to a number of geodynamic
and geochemical phenomena that have been attributed
to much deeper boundary layers.
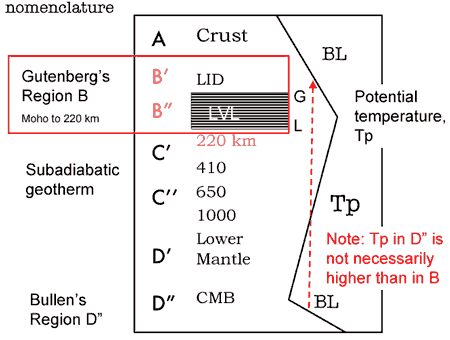
Figure 1: Nomenclature of the mantle.
The classical subdivisions of the mantle are Regions
B, C, D’ and D”. Region B constitutes the
upper boundary layer of the mantle. It is much
larger than other proposed reservoirs, such as D” and
the continental lithosphere. Gutenberg (1959) placed
the boundary between Regions B and C near 220 km. The
classical lower mantle (Region D) starts at ~1000 km,
not at 650 km. The low-velocity anisotropic layer (LLAMA)
extends from the Gutenberg discontinuity (G) to the
Lehmann discontinuity (L). A schematic potential temperature
(Tp) geotherm is shown. Because of internal heating
and secular cooling, D” may have a lower Tp than
the base of B. Region B, the most heterogeneous and
anisotropic region of the mantle, includes the seismic
lid and the LVL, which extends
from the Gutenberg or G-discontinuity (70 ± 30
km depth) to the Lehman or L-discontinuity (220 ± 20
km depth). Any point on a geotherm can be assigned
a potential temperature, which is the temperature the
point would have if adiabatically expanded to zero
pressure. The concept
of a Tp, and the ability to calculate it for any point
on a geotherm, does not require that the mantle be
adiabatic or convecting.
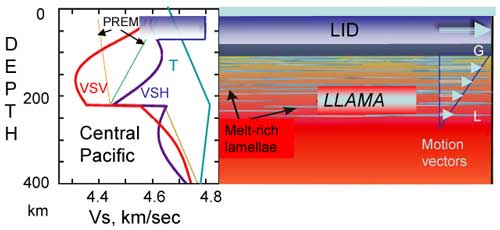
Figure 2: The LVL is anisotropic.
Horizontally propagating shear waves that are polarized
in the vertical plane, or along the symmetry axis,
travel at a velocity VSV. VSH is the shear velocity
of waves with particle motions in the symmetry plane.
Left. Seismic shear velocities, VSH and VSV, in the
central Pacific (Shapiro & Ritzwoller,
2002) and in the global reference model PREM. The steep
conduction part of the geotherm extends to > 200
km depth. Right. The laminated boundary layer model
(Kawakatsu et al., 2009) along with the interpretation
that it is a sheared layer composed of refractory and
melt-rich lamellae. The temperature increases by about
6-10°C/km with depth in a layer with a negative
Vs gradient. The minimum in VSV corresponds to the
maximum melt content. VSV is mainly sensitive to the
temperature gradient. The G discontinuity is marked
by a large decrease in shear wave velocity, typically
between 6 and 9% (e.g., Kawakatsu et al., 2009). The
L discontinuity is usually somewhat smaller. The shear
wave anisotropy between G and L is of similar magnitude.
This implies the presence of thin, low-rigidity sub-layers
or sills in the LVL.
The maximum melt content occurs at
the minimum in shear velocity, VSV, which is ~60 km
depth under ridges and 140-150 km depth in the central
Pacific (Figure 2). The strength and orientation of
the anisotropy at those depths implies a laminated
lithology with aligned melt accumulations (LLAMA).
The upper mantle surrounding Hawaii has higher than
average absolute seismic velocities for the age of
the plate, relatively low inferred temperatures and
a variable plate thickness. Ambient mantle in the boundary
layer under the central Pacific and
elsewhere, is not the same as the shallow mantle under
ridges.
The shear wave delays in the upper
220 km of the mantle, both globally and regionally,
account for most of the observed teleseismic delays
in well-instrumented well-sampled areas. Similar delays
across the Hawaiian swell, which is poorly sampled
by seismic rays, are routinely attributed to deep cylindrical
structures. The observed anisotropy and heterogeneity
of the shallow mantle in the vicinity of Hawaii yields
near-vertical, deep, plume-like structures when relative
travel-times to Hawaii are modeled in the usual way,
i.e., assuming a smooth isotropic shallow mantle. These
are artifacts, however.
Refractory remnants of ancient high-temperature
melting processes remain in the shallow mantle because
they are relatively buoyant. The fabric of the subplate
boundary layer arises from shear-bands and aligned,
melt-rich lamellae embedded in the ancient buoyant
refractory matrix. The seismic lid and the rheological
lithosphere are in the cold, top-most refractory layer
(Figure 3). The LVL is infiltrated by melts and fluids,
which are aligned by shear. The anisotropy of the LVL
is mainly due to this dynamically maintained macroscopic
fabric, which also allows melts to be retained. Melts
are released when the laminar fabric is disrupted by
steps in the lid (Figure 1), by fracture zones, and
by shear-driven upwellings. Since the fabric of the
boundary layer involves melt-rich lamellae separated
by thick depleted harzburgite layers (Figure 2), rather
than a chaotic marble-cake of multiple components,
it is easy to understand why geochemical arrays are
simple two-component mixing arrays diverging from a
common component. The major element, trace-element
and isotopic compositions of oceanic and continental
intra-plate magmas and their xenoliths do not require
sources from deeper than the boundary layer of the
upper mantle. In addition, many of the isotopic signatures
are acquired from the atmosphere, hydrosphere and sediments.
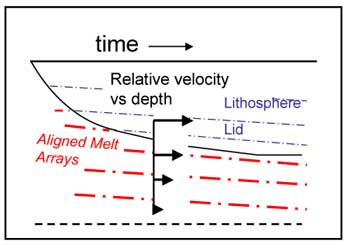
Figure 3: The LLAMA model. The
seismic lid grows at the expense of the LVL.
The lithosphere-lid system overlies aligned melt arrays,
which occur in shear-bands. The red regions are low-rigidity
lamellae. If these are melt-rich, then when they freeze
they will have high seismic velocities and the boundary
layer will then have properties similar to cratonic
mantle. Scaling relations suggest that shear-bands
and melt lenses can be tens of kilometers apart. This
macro-fabric is in addition to the grain- and vein-scale
micro-fabric, e.g., marblecake and oriented crystal
fabric.
The recycling of upper and lower continental
crust, sediments and oceanic lithosphere into the shallow
mantle, plus metasomatism by slab-derived fluids and
residual ancient lithospheric components, can account
for the entire range of chemical and isotopic signatures
in intra-plate basalts. Even the largest of the large
igneous provinces apparently originates in the shallow
mantle. The compositional heterogeneity of the mantle
is intrinsic to plate tectonics and does not require
chaotic stirring of a limited number of physically
separate mantle reservoirs into “the convecting
mantle”. There is no requirement or evidence
that any of this material circulated to the deep mantle
before being sampled.
Intimate juxtaposition of diverse
lithologies, is accomplished by boundary-layer shearing
(Figure 4). Seismic results show that most of the lithologic
heterogenity of the mantle occurs above 220 km depth.
Since seismic velocities are strongly affected by melt
and other fluids in the mafic parts of the mantle,
this suggests that this is where midplate magmas originate.
Fluids and magmas intercalated with high-velocity,
refractory components are mainly responsible for both
the heterogeneity and anisotropy of the shallow mantle.
Aligned or anastomosing arrays of melt channels result
in anisotropy of seismic velocity, viscosity, conductivity
and permeability. This unusual region of the mantle
is composed of laminated lithologies
and aligned melt arrays (LLAMA) (Figure 4). The mechanism that leads
to this fabric is lubricated lateral
advection and magma accumulation. The temperatures,
compositions and volumes of intra-plate and continental
breakup magmas are consistent with these ambient mantle
conditions and top-down tectonics. Upwellings are part
of plate tectonics but they are generally not deep-seated
or buoyant in their own right, i.e., they are not in
the form of thermal plumes. Mantle plumes are not required
by any petrological or geophysical data.
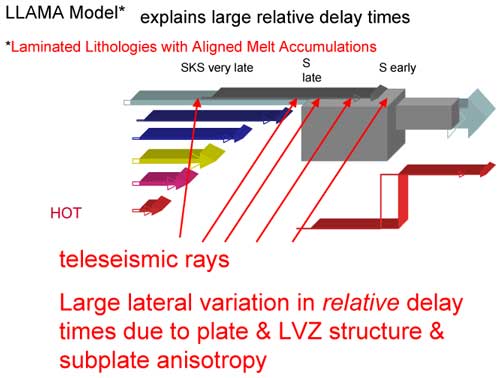
Figure 4: A variable thickness
plate moves to the right and entrains the top of
the underlying mantle, forming a shear boundary layer.
Lithospheric steps and fracture zones cause disruption
of this boundary layer. The hotter, deeper parts
of the boundary layer are almost stationary relative
to the surface. Observed teleseismic delays result
almost entirely from anisotropy and lateral variation
in the shearing horizontal boundary layer, rather
than from continuous vertical features extending
to >1000 depth (plumes). The lower portions
of LLAMA are hotter than MORB. Most samples of the
mantle, away from plate boundaries, are derived from
this sublayer. Subduction can also displace material
out of Region C but no deeper material is required
by either mass balance or geochemistry of magmas and
their xenoliths.
Laminated sublayer, laminar advection
Plate motions are decoupled from the deeper mantle
by a ~200 km thick shear boundary layer with a high
thermal gradient (Figures 3 & 4). I summarize the
more important implications of this geodynamic model
as follows:
-
Potential temperatures in
the deeper parts of LLAMA are higher than MORB
temperatures and can be higher than in D” (Figure
1).
-
The 220 ± 20 km depth
level (L) is a fundamental tectonic boundary. There
is a pronounced change in the fabric of the mantle
and the shear velocity gradient at this depth.
The mantle above this depth is by far the most
anisotropic and heterogeneous part of the mantle
(Figure 1).
-
Most mantle samples originate
above the L discontinuity (Figure 2) and most of
the geochemical components originate in the surface
layers of the Earth and are stored in LLAMA.
-
LLAMA, along with the crust, accounts for most
of the global variation in seismic travel times (Figure
4).
-
The LVL under Hawaii starts
deep. It can be converted to a craton-like boundary
layer simply by lowering the temperature and freezing
the melt-rich lamella (Figure 3).
-
That part of the oceanic upper mantle that supports
a conduction gradient is much thicker than the part
that has been cooled by conduction since the plate
formed. It is comparable to the steady-state thickness
and the thickness of cratons. The implication is
that new thermal boundary layers are emplaced atop
pre-existing ones rather than atop isothermal or
adiabatic interiors (Figure 5).
-
Melts can be extracted from
the upper boundary layer of the mantle. The “fixity” and
fluxes of intraplate volcanoes, and the associated
shallow bathymetry can all be explained by boundary
layer dynamics, with sources in and near the base
of a lubricating layer of ambient mantle, i.e.,
LLAMA (Figure 4).
-
Ambient mantle surrounding Hawaii has relatively
high seismic velocities and normal or low inferred
temperatures. Nevertheless, it is a truism that half
of the teleseismic delays to Hawaiian stations within
the local region are slower than the other half.
-
Waveform modeling, high-resolution
seismology and the central
limit theorem show that low-velocity
anomalies in the mantle are more pronounced, sharper
and less continuous than the tomographic images
on which current geochemical and geodynamic speculations
are based. Sharp boundaries are unlikely to be
caused by long-lived thermal upwellings.
-
The kind of shallow heterogeneity and anisotropy
measured by seismology, when sampled by sparse near-vertical
teleseismic rays and analyzed by the usual tomographic
methods, will yield near-vertical or tilted plume-like
structures centered under the seismic array. This
will occur wherever the array is placed, providing
misleading indications of plumes.
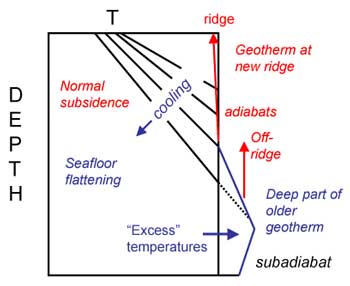
Figure 5: Schematic diagram of
the growth of a thermal boundary layer that is emplaced
atop a previously cooled part of the mantle. Mid-ocean
ridges form by the fracturing of the top part of a
mature boundary layer, which is then dragged away by
diverging plates. The boundary layer is perturbed by
the formation of a ridge (e.g., Presnall & Gudfinnsson,
2011). Mantle upwells from some shallow depth to fill
the opening crack, imposing an adiabatic gradient on
the part of the mantle above the level of upwelling,
which subsequently cools, forming a new thermal boundary
layer. The deeper parts of the boundary layer can have
higher Tp than ridge basalts or even all or part of
D”. The red arrows, projected to the surface,
give the Tp of points on the geotherm.
Summary
There is no longer any plausible evidence
or argument for deep plumes under midplate volcanoes
or for accessible lower mantle reservoirs. The widely
quoted speculations to the contrary ignore the dominant
roles of fabric and shallow boundary layers. The interpretation
of seismic anisotropy in terms of aligned macroscopic
lamellae and sills rather than as subsolidus olivine
orientation, has immediate implications for mantle
petrology and geochemistry and the locations and geometric
relations of the sources of geochemical components.
A guide to the 2009-2011 & related
literature
The message of Anderson (2010) and
the references therein is that the surface boundary
layer of the mantle, horizontal advection, and fabric
are the keys to midplate magmatism and mantle dynamics.
Recent studies have confirmed these views. It is now
possible to model boundary layer scales of mantle dynamics
(e.g., Adam et al., 2010; Schmandt & Humphreys,
2010a; Conrad et al., 2010; Stadler et
al., 2010; Faccenna & Becker, 2010; Leahy
et al., 2010; Van Wijk et al., 2010),
to explain the low Tp in D” (Schuberth
et al., 2009; Sinha & Butler, 2007),
and to map boundary layer stratigraphy with xenoliths
(Ishikawa et al., 2011). High-resolution seismic
techniques confirm the importance of Region B (Schmandt & Humphreys,
2010b; Ritsema et al., 2010; Leahy et
al.,
2010; Sun & Helmberger, 2010). The components
of oceanic basalts can all be found in the shallow mantle
(Griffin et al., 2009; Salters & Sachi-Kocher,
2010). Laboratory experiments confirm the melt segregation
mechanism for anisotropy (Kohlstedt et al.,
2009; Holtzman & Kendall,
2010). The older references in the list below are mentioned
in the figure captions or are essential for understanding
the background of LLAMA, and some of the geochemical
implications (e.g., Hart et al., 1992).
-
Adam, C., M. Yoshida, T. Isse, D. Suetsugu, Y. Fukao,
G. Barruol, (2010), South Pacific hotspot swells dynamically
supported by mantle flows, Geophys. Res. Lett., 37,
L08302.
-
-
-
Bryan, S.E., I. Ukstins Peate, D.W.
Peate, S. Self, D.A. Jerram, M.R. Mawby, J.S. Marsh,
J.A. Miller, (2010), The largest volcanic eruptions
on Earth, Earth-Science Reviews, 102,
207-229.
-
Cañón-Tapia,
E., (2010), Origin of large igneous provinces:
The importance of a definition, in Cañón-Tapia,
E., and Szakács, A., eds., What Is a
Volcano?,
Geol. Soc. Am. Special Paper 470, 77–101,
doi: 10.1130/2010.2470(06).
-
Conrad,
C.P., Benjun Wu, E.I. Smith, T.A. Bianco, A. Tibbetts,
(2010), Shear-driven upwelling induced by lateral viscosity
variations and asthenospheric shear: A mechanism for
intraplate volcanism, PEPI, 178, 162-175.
-
Dasgupta,
R., M.G. Jackson, C.-T. A. Lee, (2010), Major element
chemistry of ocean island basalts–conditions
of mantle melting and heterogeneity of mantle source, Earth
Planet. Sci. Lett., 289,
377-392.
-
Faccenna, C. and T.W.
Becker, (2010), Shaping mobile belts by small-scale
convection, Nature, 465, 602-605, doi:10.1038/nature09064.
-
-
Griffin,
W.L., S.Y. O’Reilly, J.C. Afonso, and
G.C. Begg, (2009), The composition and evolution
of lithospheric mantle: a re-evaluation and its
tectonic implications. J.
Petrology, 50, 1185-1204.
-
Gutenberg,
B. (1959). Wave velocities below the Mohorovicic
discontinuity, Geophys.
J. Royal Astr. Soc., 2, 348-352.
-
Hamilton,
W.B., (2011), Plate tectonics began in Neoproterozoic
time, and plumes from deep mantle have never operated, Lithos,
doi:10.1016/j.lithos.2010.12.007
-
Hart,
S.R., E.H. Hauri, L.A. Oschmann, and J.A. Whitehead,
(1992), Mantle plumes and entrainment–isotopic
evidence, Science, 256, 517–520.
-
Holtzman,
B.K., Kohlstedt, D.L., Zimmerman, M.E., Heidelback,
F., Hiraga, T. and Hustoft, J., (2010). Melt segregation
and strain partitioning: Implications for seismic
anisotropy and mantle flow, Science, 301,
1227-1230.
-
Holtzman,
B.K., and J.-M. Kendall, (2010), Organized melt, seismic
anisotropy, and plate boundary lubrication, Geochem.
Geophys. Geosyst., 11, Q0AB06, doi:10.1029/2010GC003296.
-
Ishikawa, A., D.G. Pearson, C.W. Dale,
(2011), Ancient Os isotope signatures from
the Ontong Java Plateau lithosphere: Tracing
lithospheric accretion history, Earth Planet.
Sci. Lett., 301,
159-170.
-
Kawakatsu,
H., Kumar, P., Takei, Y., Shinohara, M., Kanazawa,
T, Araki, E. & Suyehiro,
K. (2009), Seismic evidence for sharp lithosphere-asthenosphere
boundaries of oceanic plates. Science, 324,
499-502; doi:10.1126/science.1169499.
-
Kohlstedt,
D.L., Zimmerman, M.E.
& Mackwell, S.J., (2009), Stress-driven melt
segregation in partially molten rocks, J. Petrology, doi:10.1093/petrology/egp043.
-
Leahy,
G.M., J.A. Collins, C.J. Wolfe, G. Laske and S.C. Solomon,
(2010), Underplating of the Hawaiian Swell: evidence
from teleseismic receiver functions, Geophys. J.
Int., 183, 313–329,
2010, doi: 10.1111/j.1365-246X.2010.04720.x.
-
-
Ritsema, J., A. Deuss, H.J. van Heijst
and J.H. Woodhouse, (2010), S40RTS: a degree-40 shear-velocity
model for the mantle from new Rayleigh wave dispersion,
teleseismic traveltime and normal-mode splitting
function measurements, Article first published online:
14 Dec 2010, doi: 10.1111/j.1365-246X.2010.04884.x
-
Salters,
V.J.M., A. Sachi-Kocher, (2010), An ancient metasomatic
source for the Walvis Ridge basalts, Chem. Geol., 273,
151-167.
-
Schmandt,
B., and E. Humphreys, (2010a), Complex subduction
and small-scale convection revealed by body-wave
tomography of the western United States upper
mantle, Earth Planet. Sci. Lett., 297,
435-445.
-
Schmandt, B.,
and Humphreys, E., (2010b), Seismic heterogeneity
and small-scale convection in the southern California
upper mantle, Geochem.
Geophys. Geosyst., 11, Q05004.
-
Schuberth, B.S.A.,
H.P. Bunge, G. Steinle-Neumann, C. Moder, and J. Oeser,
(2009). Thermal versus elastic heterogeneity in high-resolution
mantle circulation models with pyrolite composition:
High plume excess temperatures in the lowermost mantle. Geochem.
Geophys. Geosyst., 10, Q01W01; doi:10.1029/2008GC002235.
-
Sinha,
G. and S.L. Butler, (2007), On the origin and significance
of subadiabatic temperature gradients in the mantle, J.
Geophys. Res., 112, B10406; doi:10.1029/2006JB004850.
-
Shapiro,
N.M. and M.H. Ritzwoller, (2002), Monte-Carlo inversion
for a global shear velocity model of the crust
and upper mantle, Geophys.
J. Int., 151, 88-105.
-
Stadler,
G. et al. (2010), The dynamics of plate tectonics
and mantle flow: From local to global scales, Science, 329,
1033-1038, doi: 10.1126/science.1191223.
-
Sun,
Daoyuan and D. Helmberger, (2010), Upper-mantle structures
beneath USArray derived from waveform complexity, Geophys.
J. Int.,
published online: 30 Nov 2010, doi: 10.1111/j.1365-246X.2010.04847.x.
-
Van
Wijk, J.W., W.S. Baldridge, J. van Hunen, S. Goes,
R. Aster, D. Coblentz, S.P. Grand, and J. Ni, (2010),
Small-scale convection at the edge of the Colorado
Plateau: Implications for topography, magmatism,
and evolution of Proterozoic lithosphere, Geology, 38,
611 614, doi:10.1130/G31031.1.
-
Willbold,
M. and A. Stracke, (2010), Formation of enriched mantle
components by recycling of upper and lower continental
crust, Earth Planet. Sci. Lett., 297, 188-197.
last updated 16th
March, 2011 |