S.
D. King1 &
H. L. Redmond2
1Department of Geosciences, Virginia
Tec, 4044 Derring Hall (0420, Blacksburg, VA 24061
2Department
of Earth and Atmospheric Sciences, 550 Stadium Mall
Drive, Purdue University, West Lafayette, IN 47907-2051
1sdk@vt.edu,
2redmondh@purdue.edu
Tharsis rise is
one of the most prominent features on the surface
of Mars and dominates the global topography and areoid
on Mars (Figure 1). The broad topographic swell, more
than 6,500 kilometers wide, includes the shield volcanoes
Arsia Mons, Pavonis Mons, Ascraeus Mons, Alba Patera
and Olympus Mons, the largest known shield volcano
in the solar system, towering 21.3 km above the Martian
surface. To get a sense of the size of Olympus Mons
one should compare this height to Mauna Loa, Hawaii,
which rises 8.9 km above the ocean floor. The high-resolution
Mars Orbiting Laser Altimeter (MOLA) topography (Figure
1) provides a striking view of the relationship between
the Tharsis province and the shield volcanoes (Smith
et al., 1999). Of particular note, Olympus Mons
sits off the western edge of the Tharsis rise rather
than sitting on the flank, as in previous topographic
models (c.f., Wu, USGS Map, 1991; Smith
et al., 1999). Even so, there remains an assumed
link between Olympus Mons and Tharsis rise in many
researchers minds because of the spatial proximity
of these features.
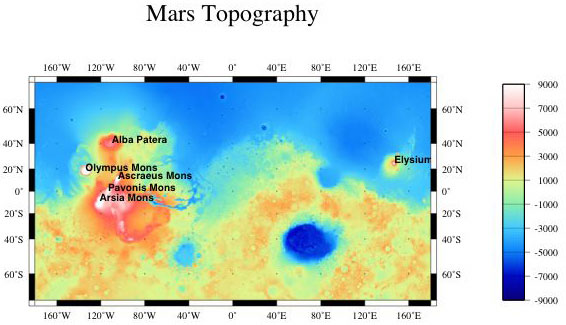
Figure 1: Martian
topography taken from the Mars Orbiting Laser
Altimeter (MOLA). This image was produced using
an Initial Experiment Gridded Data Record on
the Planetary Data System (PDS) geoscience node.
The resolution is 16 pixels per degree.
The MOLA topography
provides a detailed view of Tharsis rise, clearly
showing that the Tharsis region consists of two broad
rises; the southern rise (from 50°S to 20°N)
which includes the Tharsis Montes (Arsia, Pavonis,
and Ascraeus Mons) and the more northern rise (from
~20°N to 60°N) which is dominated by Alba
Patera. The southern rise contains heavily cratered
regions of Noachian age (4400–3600 Ma). The
broad, elevated, ancient terrains are consistent with
the view that some of the uplift of Tharsis is tectonic
and may not entirely be volcanic construction (c.f.,
Banerdt et al., 1982; Solomon & Head,
1982; Phillips et al., 1990; Tanaka et
al., 1991; Schultz & Tanaka, 1994).
Martian
Geological Periods |
Noachian |
4.6-3.5
Ga |
Hesperian |
3.5-1.8
Ga |
Amazonian |
1.8
Ga - present |
Given the popularity
of the Plume Hypothesis (Wilson, 1963;
Morgan, 1971) as an explanation for swells
and intraplate volcanism on Earth, it is not surprising
that a plume has been proposed as the formation
mechanism of Tharsis rise (c.f., Zuber,
2001; Schubert et al., 2001, and references
therein). A recent modification of the plume model
for Tharsis is that multiple plumes feed (or once
fed) each volcano (Kiefer, 2003). Because
the natural convection pattern in a spherical shell
forms cylindrical, plume-like upwellings, it seems
reasonable to assume that mantle plumes exist, or
once existed, on other terrestrial planets (Schubert
et al., 2001). However, a number of lines of
evidence are leading to the conclusion that Tharsis
is not underlain by a plume now (e.g.,
Lowry & Zhong, 2003; Zhong &
Roberts, 2003) and others go further to speculate
that it may never have been underline by a plume
(e.g., Stegman et al., 2003).
Two lines of evidence
are generally used to question the plume explanation
of Tharsis topography: heatflow and mantle energetics;
and topography and areoid (i.e., the Martian geoid).
Note that there is a variety of opinions ranging through:
-
there
is (must be) an entirely non-plume explanation for
the formation and evolution of Tharsis rise,
-
Tharsis
rise formed via a plume head interacting with the
lithosphere in the late Noachian (~3500 Ma) but
all that remains now is the volcanic rise (i.e.,
there is very little or no dynamic contribution
to Tharsis rise), and
-
Tharsis
rise is (could be) actively underlain by one (or
more) mantle plume(s) (Kiefer, 2000; 2003;
Redmond & King, in press) even though
some (much) of the topography is due to volcanic
construction
Heatflow
and mantle energetics
Mars has a larger
surface area to volume ratio than Earth because the
radius of Mars is about half that of Earth. This could
allow for more rapid heat loss and cooling of the Martian
interior as compared with Earth. The complicating factor
is that Earth has mobile plates at the surface that
significantly aid cooling, but Mars has a stagnant lid
(c.f., Gurnis, 1989; Solomatov & Moresi,
2000). If Mars ever had plate tectonics, it has now
ceased (Sleep, 1994). In addition, crustal
differentiation on Mars appears to have been more efficient
(Spohn, 1991; Schubert et al., 1992)
and a significant fraction of the incompatible, heat-producing
elements are thought to be near the surface of Mars
where heat loss would be dominated by conduction (Schubert
et al., 2001).
If the heatflow from
the core is small, as some thermal history models suggest
(e.g. Hauck & Phillips, 2002), then it
may be difficult for a plume to exist at present and
the plume mode of convection may have shut off early
in Mars history. The evidence for a magnetic field –
and apparently early shutoff of the magnetic field about
4 Gya – has led to the idea that the core dynamo
may have shut off early in Mars history (Acuna et
al., 1999). The argument is that the core dynamo
shut off as the core cooled. The problem with this argument
is that the dynamo on Earth is most likely powered by
compositional as opposed to thermal, buoyancy (Braginsky
& Roberts, 1995; Buffett et al., 1996;
Schubert et al., 2001). So it isn't clear that
the magnetic field and core heatflow are directly related.
The lack of seismic
instrumentation on Mars is a significant limitation.
The exact size of the core, the presence or absence
of an inner core, whether the core is completely solid
or partially molten, the velocity structure of the mantle
and the structure of the Moho, all would be potentially
resolvable with seismic instruments on Mars. Unfortunately,
seismometers always seem to be cut from Mars missions.
Areoid
and topography over Tharsis Rise
Zhong & Roberts
(2003) and Lowry & Zhong (2003) have shown
that a significant amount of the support of the Tharsis
topographic anomaly can be explained by a volcanic structure
modifying the lithosphere. Approximately 75% of the
areoid and topographic profiles over Tharsis can be
explained by crustal thickness and surface volcanic
construction (c.f., Solomon & Head, 1982;
Zuber, 2001; Zhong, 2002; Lowry
& Zhong, 2003; Zhong & Roberts,
2003). Given the crustal thickness anomalies, some Mars
researchers question whether a mantle plume could be
active on Mars presently and speculate that recent volcanism
on Tharsis may have formed by a non-plume mechanism
(e.g., King
& Ritsema,
2000). Redmond & King (in press) show
that it is possible to produce a mantle plume that has
a small enough topography and geoid anomaly to explain
the residual topography and areoid (the part not explained
by the 75% from the Zhong & Roberts (2003)
study). Because gravity and dynamic topography models
are non-unique, it seems likely that gravity and topography
will not be able to definitively rule out a present-day
plume without additional information (such as the seismic
structure of the Tharsis swell).
One first-order observation
of the long-wavelength areoid and topography is that
there is a striking spherical-harmonic degree-2 order-2
pattern (i.e., two equatorial highs almost 180 degrees
apart) (Figure 2). Phillips et al. (2001) have
shown that this can be modeled considering elastic stresses
in the lithosphere from a single topographic load at
Tharsis rise. While it has not been demonstrated, we
strongly suspect that this is independent of the source
of the load, that is dynamic uplift from a buoyant mantle
source would also lead to the same degree 2-2 pattern
in the areoid.
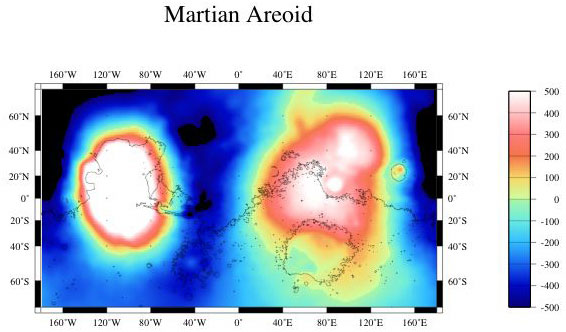
Figure 2: Martian
Areoid from model JOD75E60. Taken from the Planetary
Data System (PDS) geoscience node. Black line is
the zero contour of the topography from Figure
1. This approximates the location of the crustal
dichotomy.
In
defense of plumes on Mars?
Even though Mars is
smaller than Earth and the Martian mantle is likely
to be convecting less vigorously than Earth's mantle,
convection calculations in 3D spherical geometry with
the appropriate parameters are time-dependent (Schubert
et al., 1990). In these calculations plumes migrate
relative to each other with time (Schubert et al.,
2001), particularly early in Mars' thermal evolution.
Schubert et al. (1990) point out that during
the later stages of the calculations, the mantle settles
into a stable, two-plume planform. However, earlier
in the same calculations they observe six or more plumes.
These calculations are difficult to reconcile with the
observation that there has been one dominant volcanic
feature on Mars that was active from at least 3 Gyr
before present until about 40 Mya and that this volcanic
feature has remained stationary with respect to the
crust. The volcanic activity at Tharsis and Elysium
could possible indicate two plumes (following the plume
hypothesis). However, the early stages of all Martian
convection calculations have more than two plumes. In
these calculations, plumes are always scattered, and
not clustered as they would have to be to explain Tharsis.
Why the additional plumes that appear early in the convection
calculations never developed significant volcanic features
remains unanswered.
Harder & Christensen
(1996) and Harder (1998) show that a perovskite-magnesiowustite
layer at the base of the Martian mantle will suppress
short-wavelength instabilities from the bottom thermal
boundary layer, making it is possible to produce a single
upwelling plume. It requires more than 4 Ga of evolution
of the calculation before the convective flow settles
into a one-plume planform, however, which begs the question
of why the early volcanic activity appears to adopt
the single plume planform. Harder (1998) estimates
the depth of the perovskite phase transition on Mars
to be roughly 1900 km, which requires the core to be
smaller than 45% of the planetary radius. Without the
stabilizing effect of the perovskite layer at the base
of the mantle, Schubert et al. (1990) demonstrate
that a planform with six to twelve upwelling plumes
is likely. As the planet cools, a planform with two
stable upwellings can be achieved without the deep perovskite
layer (e.g., Schubert et al., 2001).
The
Crustal Dichotomy
The crustal dichotomy
may be one of the oldest features on Mars. The southern
hemisphere of Mars is highly cratered and approximately
4.5 km higher in mean elevation than the smooth and
relatively-low-cratered surface northern hemisphere.
A larger number of buried craters that were not visible
in previous spacecraft images have been identified in
the MOLA topography (Frey et al., 2002). Thus,
while the crater age of the southern hemisphere has
always been known to be old, it is now clear that the
northern hemisphere is also old with the bulk of the
older, Martian crust emplaced during the Noachian (Schubert
et al., 2001). The heavily cratered, older crust
of the northern hemisphere has since been overlaid by
lava flows dating back to the late Hesperian up through
the late Amazonian (Schubert et al., 2001)
forming the smooth, northern plains observed today.
Logically it follows that the crustal dichotomy is also
an old feature. Tharsis rise straddles the crustal dichotomy
of Mars and this leads to the question of whether the
dichotomy played a role in the formation and evolution
of Tharsis.
The elevation difference
between the northern and southern hemispheres requires
a thinner crust in the north. The lack of correlation
between the gravity field (Figure 2) and the dichotomy
boundary (Figure 1) is consistent with the hypothesis
that the crustal dichotomy is an ancient feature (see
Kaula (1967) for the discussion of the lack
of correlation between the gravity field and continents
on Earth). Hence a relatively sharp discontinuity in
crustal thickness must have been maintained thoughout
much of Martian history. Thus, mass variations in the
Martian crust are assumed to be due to changes in crustal
thickness of a nearly uniform density crust (Zuber
et al., 2000). The analogy of passive margins on
Earth might lead one to think that there is a compositional
difference. However, there is no indication that there
has been silicic volcanism on Mars. The variation of
crustal thickness over a short length-scale, as suggested
by the sharp dichotomy boundary, is not unlike that
at a passive margin or craton boundary. Edge-driven
convection may play a role in some hotspots on
Earth (King
& Anderson,
1998; 1998; King
& Ritsema,
2000) and the dichotomy boundary may be an ideal
situation for edge-driven convection.
In a lab experiment
performed by Wenzel et al. (2004), it was shown
that if a thick insulating layer is placed over half
of a tank containing a two-layered mantle-like system,
an upwelling will develop early under the insulating
layer and persist for billions of years. Although the
upwelling forms beneath the layer and not at the edge,
where Tharsis lies, it is still strongly suggestive
that the crustal dichotomy may have played a role in
the formation of the Tharsis rise.
Conclusion
At present it is difficult
to unequivocally favor or rule out any mechanism for
the formation of Tharsis rise and the shield volcanoes
of Mars. However, several things are clear:
-
The
Tharsis province comprises at least two separate
rises, and while both have clear volcanic construction
features, some of the uplift, particularly on the
southern rise, may be of tectonic and not volcanic
construction;
-
It
is unclear why one (and only one) unusually-large
volcanic region formed on Mars and how this is linked
to mantle dynamics, because most mantle convection
calculations suggest that there should be multiple,
mobile upwelling plumes early in Mars history;
-
There
is an interesting, maybe causative, spatial association
between the crustal dichotomy of Mars and the Tharsis
region.
There are some aspects
of Tharsis rise that are generally consistent with a
plume model, and some that are not. Knowing the seismic
velocity structure of Tharsis rise and the Martian mantle
below it would be very useful. However, it is difficult
to envision the kind of seismic experiment that has
been performed at a number of hotspots on Earth being
possible on Mars in the near future. Even first-order
unknowns, such as the radius of the core, whether there
is an inner core, and a 1D velocity model for the Martian
mantle would be extraordinarily helpful.
References
-
Acuna,
M. H., J. E. P. Connerney, N. F. Ness, R. P. Lin,
D. Mitchell, C. W. Carlson, J.
McFadden, K. A. Anderson, H. Reme, C. Mazelle, D.
Vignes, P. Wasilewski, and P. Cloutier, Global distribution
of crustal magnetization discovered by the Mars
Global Surveyor MAG/ER experiment, Science, 284, 790-793, 1999.
-
Banerdt,
W.B., R.J. Phillips, N.H. Sleep, and R.S. Saunders,
Thick shell tectonics on one-plate
planets: Applications to Mars, J. Geophys. Res.,
87, 9723-9733, 1982.
-
Banerdt,
W.B., M.P. Golombek, and K.L. Tanaka, Stress and
tectonics on Mars, in Mars, edited by H.H. Kieffer, B.M. Jakosky, C.W. Snyder, and M.S. Matthews,
pp. 249-297, Univ. of Arizona
Press, Tucson, 1992.
-
Braginsky, S. I. and P. H. Roberts, Equations governing
convection in Earth's core and the geodynamo, Geophys.
Astrophys. Fluid Dyn., 79,
1-97, 1995.
-
Buffet, B. A., H. E. Huppert, J. R. Lister, and A.
W. Woods, On the thermal evolution of the Earth's
core, J. Geophys. Res., 101, 7989-8006, 1996.
-
Frey, H. V., J. H. Roark, K. M. Shockey, E. L. Frey,
and S. E. H. Sakimoto, Ancient lowlands on Mars,
Geophys. Res. Lett., 29, 10.1029/2001GL013832, 2002.
-
Gurnis, M., A reassessment of the heat transport by
variable viscosity convection with plates and lids,
Geophys. Res. Lett., 16,
179-182, 1989.
-
Harder, H., Phase transitions and the three-dimensional
planform of thermal convection in the Martian mantle,
J. Geophys. Res., 103, 16,775-16,797, 1998.
-
Harder, H. and U. Christensen, A one-plume model of
Martian mantle convection, Nature,
380, 507-509, 1996.
-
Hartmann,
W.K., M. Malin, A. McEwen, M. Carr, L. Soderblom,
P. Thomas, E. Danielson,
P. James, and J. Veverka, Evidence for recent volcanism
on Mars from crater counts, Nature,
397, 586-589, 1999.
-
Hauck,
S.A. and R.J. Phillips, Thermal and crustal evolution
of Mars, J. Geophys. Res.,
107, 5052, doi:10.1029/2001JE001801, 2002.
-
Kaula,
W. M., Theory of statistical analysis of data distributed
over a sphere, Rev. Geophys., 5, 83-107, 1967.
-
Kiefer,
W. S., Magma production and mantle convection on
Mars, Lunar Planet. Sci., XXXI, abstract 1527, 2000.
-
Kiefer,
W.S., Melting in the Martian mantle: Shergottite
formation and implications for present-day
mantle convection on Mars, Meteor. Planet. Sci.,
38, 1815-1832, 2003.
-
King,
S.D. and D.L. Anderson, An alternative mechanism
to flood basalt formation, Earth Planet. Sci. Lett., 136, 269-279, 1995.
-
-
-
Lowry,
A.R. and S. Zhong, Surface loading versus internal
loading of the Tharsis Rise, Mars,
J. Geophys. Res.,
108, 5099, doi:10.1029/2003JE002111,
2003.
-
Morgan,
W.J., Convection plumes in the lower mantle, Nature,
230, 42-43, 1971.
-
Phillips,
R. J., N. H. Sleep and W. B. Banerdt, Permanent
uplift in magmatic systems with
application to the Tharsis region of Mars, J.
Geophys. Res., 95,
5089-5100, 1990.
-
Phillips,
R. J., M. T. Zuber, S. C. Solomon, M. P. Golombeck,
B. M. Jakosky, W. B. Banerdt,
R. M. Williams, B. Hynek, O. Aharonson, and S. A.
Hauck II, Ancient geodynamics
and global-scale hydrology on Mars, Science,
291, 2587-2591, 2001.
-
Redmond,
H. L. and S. D. King, A numerical study of a mantle
plume beneath the Tharsis
Rise: Reconciling dynamic uplift and lithospheric
support models, J. Geophys.
Res., in press, 2004.
-
Schubert, G. Bercovici, D. and G.A. Glatzmaier, Mantle
dynamics in Mars and Venus: Influence of an immobile
lithosphere on three-dimensional mantle convection,
J. Geophys. Res.,
95, 14105-14129, 1990.
-
Schubert, G., D. L. Turcotte, and P. Olson, Mantle
Convection in the earth and planets,
Cambridge University Press, 2001.
-
Schubert,
G., S.C. Solomon, D.L. Turcotte, M.J. Drake, and
N.H. Sleep, Origin and thermal
evolution of Mars, in Mars,
edited by H. H. Kieffer, B.M. Jakosky, C.W. Snyder,
and M.S. Matthews, pp. 147-183, Univ. of Arizona
Press, Tucson, 1992.
-
Schultz,
R. A. and K. L. Tanaka, Lithospheric-scale buckling
and thrust structures on Mars:
The Coprates rise and the south Tharsis ridge belt,
J. Geophys. Res., 99, 8371-8385,
1994.
-
Sleep,
N.H., Martian plate tectonics, J. Geophys. Res.,
99, 5639-5655, 1994.
-
Smith, D.E., Zuber, M.T., Solomon, S.C., Phillips,
R.J., Head, J.W. et al., The global topography of
Mars and implications for surface evolution, Science, 284, 1495- 1503, 1999.
-
Solomatov, V. S. and L. N. Moresi, Scaling of time-dependent
stagnant lid convection: Application to small-scale
convection on the Earth and other terrestrial planets,
J. Geophys. Res.,
105, 21,795-21,818, 2000.
-
Solomon, S.C. and J.W. Head, Evolution of the Tharsis
province of Mars: The importance of heterogeneous
lithospheric thickness and volcanic construct, J.
Geophys. Res., 87, 9755-9774, 1982.
-
Spohn,
T., Mantle differentiation and thermal evolution
of Mars, Mercury and Venus, Icarus, 90, 222-236, 1991.
-
Stegman, D., M. Jellinek, M. Richards, M. Manga, and
J. Baumgardner, Theories of Mars' interior: An alternative
to plumes as the origin of Tharsis, Eos Trans.
AGU, 84(46),
Fall Meet. Suppl., Abstract SS12F-05, 2003.
-
Tanaka, K. L., M. P. Golombeck, and W. B. Banerdt,
Reconciliation of stress and structural histories
in the Tharsis region of Mars, J. Geophys. Res.,
96, 15,617-15,633,
1991.
-
Wilson, J. T., Evidence from islands on the spreading
of ocean floors, Nature,
197, 536-538, 1963.
-
Zhong,
S., Effects of lithosphere on the long-wavelength
gravity anomalies and their implications
for the formation of the Tharsis rise on Mars, J.
Geophys. Res., 107, 5054, doi:10.1029/2001JE001854,
2002.
-
Zhong,
S. and J.H. Roberts, On the support of the Tharsis
Rise on Mars, Earth Planet. Sci.
Lett., 214, 6765, doi:10.1016/S0012-821X(03)00384-4,
2003.
-
Zuber, M. T., The crust and mantle of Mars, Nature, 412, 220-227, 2001.
-
Zuber, M. T., S. C. Solomon, R. J. Phillips, D. E.
Smith, G. L. Tyler, O. Aharonson, G. Balmino, W.
B. Banerdt, J. W. Head, C. L. Johnson, F. G. Lemoine,
P. J. McGovern, G. A. Neumann, D. D. Rowlands, and
S. Zhong, Internal structure and early thermal evolution
of Mars from Mars Global Surveyor topography and
gravity, Science,
287, 1788-1793, 2000.
-
Wenzel, M. J., M. Manga, and A. M. Jellinek, Tharsis
as a consequence of Mars dichotomy and layered
mantle, Geophys. Res. Lett., 31, L04702, doi:10.1029/2003GL019306,
2004.
-
Wu, S. S. C., USGS Map I-2160,
1991.
last updated September 12th,
2004 |