 |
 |
Impact-induced
Martian mantle plumes: Origin of Tharsis |
|
|
|
Introduction
Tharsis is one of the most prominent
features on Mars. Volcanism and tectonism associated
with the plateau by far exceed the levels of activity
in other areas of the planet. This monopolar distribution
of tectonism and volcanism led to the suggestion
that the planform of mantle convection on Mars
is dominated by a single, long-lived, thermal
plume originating at the core-mantle boundary
similar to a terrestrial plume but much larger
(Hartmann, 1973; Carr, 1974;
Phillips & Ivins, 1979). Deep mantle
mineralogical phase transformations are capable
of stabilizing a one-plume pattern of convection
(Weinstein, 1995; Harder & Christensen,
1996; Breuer et al., 1998; Spohn
et al., 1998; Harder, 2000). However,
although the conventional plume model explains
some features of Tharsis, there are both observational
and theoretical reasons to consider alternatives.
- The plume model has not reproduced Tharsis
development on timescales consistent with
observations (Banerdt & Golombek,
2000; Phillips et al., 2001; Johnson
& Phillips, 2003; Zuber,
2001).
- The plume model only accounts for dynamic
support of Tharsis. Crustal thickening (Zuber
et al., 2000) and observations of layered
sequences of rock in the walls of Valles Marineris
(McEwen et al., 1999) suggest that
constructional volcanism is a major contributor
to Tharsis elevation (Solomon & Head,
1982). Also, a recent analysis suggests that
Tharsis is predominantly supported by crustal
thickening and lithospheric flexure while
a thermal plume would contribute only a fraction
to the present-day topography and areoid (Zhong,
2002; Lowry & Zhong, 2003; Zhong
& Roberts, 2003).
- The plume hypothesis implies an actively
convecting mantle and a sufficiently large
heat flux from the Martian core. However,
in the absence of plate tectonics, Martian
mantle convection can be very sluggish (Grasset
& Parmentier, 1998; Reese et
al., 1998; 2002; Solomatov &
Moresi, 2000). Scaling relationships
(Solomatov & Moresi, 2000) for
olivine rheology (Karato & Wu,
1993) indicate that a wet mantle convects
only in a narrow subsolidus temperature range
while a dry mantle is convectively stable
even above melting temperatures. Widespread
melting would, in turn, differentiate heat
producing elements (Turcotte, 1989;
Spohn, 1991; Schubert et al.,
1992), increase viscosity (Karato,
1986), and produce compositional stratification
(Zaranek & Parmentier, 2004)
all of which tend to suppress convection.
- Rapid development of a conventional thermal
plume at the core-mantle boundary after planetary
formation could be problematic because of
mantle heating. Although a transient episode
of early surface recycling (Sleep,
1994) can facilitate mantle cooling, the duration
might be short (Tajika & Sasaki,
1996) and the subsequent transition to stagnant
lid convection would result in mantle heating
shutting off any core heat flux and associated
plume activity (Nimmo & Stevenson,
2000). The liquid state of the Martian core
recently inferred from solar tidal deformation
is consistent with such a possibility (Yoder
et al., 2003).
- Geochemical evidence also argues against
vigorous mantle mixing. Martian meteorite
Hf/W and Sm/Nd systematics suggest that core
and crust formation were contemporaneous and
occured within ~30 Myr of planet formation
(Harper et al., 1995; Lee &
Halliday, 1997; Halliday et al.,
2001; Kleine et al., 2004) and also
indicate limited large-scale convective mixing
of the upper mantle. Survival of isotopic
heterogeneity in the Martian upper mantle
since the time of core formation and early
vigorous convection are difficult to reconcile
(Zuber, 2001). Large variations in
Sm/Nd and Lu/Hf ratios among shergottites
also suggest a heterogeneous mantle which
retains an isotopic signature of initial differentiation
(Albarede et al., 2000). Finally,
cessation of Xe degassing early in Mars history
suggests that large scale mantle magmatism,
and presumably vigorous convection, have been
limited since ~300 Myr (Marty & Marti,
2002) after formation.
|
Alternative
hypothesis: Impact plume
An alternative hypothesis is
that Tharsis could have experienced a large impact
early in Martian history (Schultz & Glicken,
1979; Schultz, 1984; Schultz &
Frey, 1990). Recent studies suggest that
this hypothesis is plausible from a geodynamical
point of view (Reese et al., 2002; 2004).
Large impacts at the end of planetary formation
are a statistically inevitable consequence of
accretional dynamics (Wetherill, 1985,1990;
Weidenschilling et al., 1997; Chambers
& Wetherill, 1998; Agnor et al.,
1999) and result in melting beyond the excavation
zone producing an intact melt region with radius
of the order of several impactor radii (Melosh,
1990; Tonks & Melosh, 1992;1993;
Pierazzo et al., 1997).
Three major processes following
impact-generation of an intact melt volume are
crystallization, isostatic adjustment and melt
percolation. Melt distribution in the impact-heated
region varies strongly, from superliquidus conditions
near the surface to subsolidus conditions deeper
in the mantle. Cooling and crystallization of
regions with the highest degree of melting to
about 60% crystal fraction can be very fast, on
the scale of 1000 years (Solomatov, 2000).
For Mars, isostatic adjustment of the region with
melt fraction varying from 40% to 0% and melt
percolation through the partially crystallized
matrix are much slower processes (Reese
et al.,
2004).
A qualitative description of
the process is as follows. Fast initial crystallization
produces a region with melt fraction varying from
about 40% near the surface to 0% several hundred
kilometers below the surface. Because of its positive
buoyancy, this region floats up. Hot, partially
molten material rises and spreads out on the surface
of the planet and is replaced by colder, less
molten material from below. All processes slow
down as the melt fraction and driving density
difference decrease and the viscosity increases.
At some point, the dynamics merge
with the solid state convective evolution of the
planet. Further upwelling results in decompression
melting and production of compositional buoyancy
(through mantle depletion and melt retention).
The end result is a long-lived (compared to initial
dynamical timescales) localized mantle upwelling
and associated magmatism – an "impact
plume". Production of Tharsis by an impact
plume requires neither globally occurring convection
nor generation of conventional plumes at the core-mantle
boundary. |
Numerical simulation
A fully three-dimensional spherical
shell simulation was used to study thermal, magmatic,
and compositional aspects of Martian impact-plume
evolution (Reese
et al.,
2004). A strongly temperature-dependent exponential
viscosity law and rigid upper surface boundary
condition is assumed ensuring stagnant lid convection
(Solomatov & Moresi, 2000). There
is no radiogenic internal heating. The average,
pre-impact, mantle temperature is 1800 K with
a fixed surface temperature of 220 K. The initial
intact melt region is hemispherical with radius
R related to impactor size and velocity (see,
e.g., Tonks & Melosh, 1993). For
the case presented here, R=1500 km, corresponding
to an impactor radius of ~900 km (Reese et al.,
2004). After fast initial crystallization it is
assumed that this region has a uniform thermal
anomaly of 300 K. Because any core heat flux was
probably short lived (Nimmo & Stevenson,
2000), the bottom boundary is assumed to be insulating
throughout evolution. The initial cold boundary
layer thickness is resolution-limited to ~300
km. For these initial conditions, there is a partially
molten sublithospheric layer ~500 km thick. This
layer is compositionally buoyant due to the presence
of melt and the fractional density difference
is assumed to be ~2%. Subsequent decompression
melting due to upwelling mantle results in the
same compositional buoyancy (via mantle depletion
and melt retention). Only dry melting below 8
GPa is considered.
Results
The initial upwelling velocity
decays with time from a maximum that scales
inversely with interior viscosity. Subsequent
evolution depends on interior viscosity.
For high interior viscosity (1023
Pa s), thermal evolution is dominated by
conduction. The stagnant lid thickens conductively
but remains convectively stable. Interior
velocities are very low and approximately
constant throughout evolution. For low interior
viscosity (1021 Pa s), lid thickening
leads to development of small scale instabilities
at the lid base. The mantle heat flux and
velocity decay with time as the spherical
shell cools (Figure 1).
Figure 1. Convective
mantle heat flux (left) and maximum interior
velocity (right) as a function of time (heavy
lines). For the high interior viscosity
case (1023 Pa s), the heat flux due to conductive
cooling of a half space is also shown (thin
line). For the low interior viscosity case
(1021 Pa s), the heat flux and cold plume
velocity (thin lines) are shown for a parameterized
convection calculation based on time-dependent
stagnant lid convection scaling laws (Solomatov
& Moresi, 2000). |
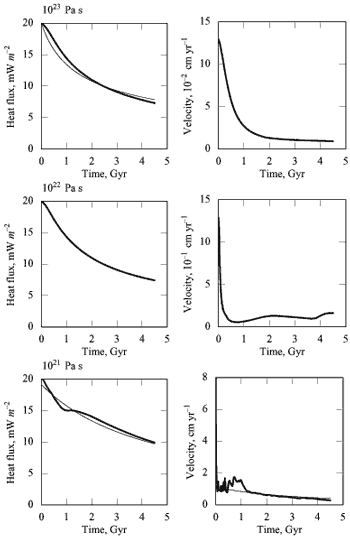
|
The duration of the magmatic
episode depends on interior mantle viscosity.
Buoyant, depleted mantle which has undergone melting
spreads out at the bottom of the viscous lid.
For all cases, melt production decays with time
from an initial maximum to very low levels. As
interior viscosity decreases, the decay rate and
total melt volume decrease and increase, respectively.
The surface distribution of volcanism is directly
related to the radially integrated melt fraction.
Since magma transport to the surface in the stagnant
lid regime is poorly understood, no attempt is
made to discriminate between intrusive and extrusive
flux. Instead, the total melt volume per unit
surface area (crustal thickness if all melt contributes
to crustal growth) is calculated. In all cases,
widespread volcanism is suppressed by lid thickening,
and volcanism is concentrated in the impact plume
region. For low interior viscosities, there are
two spatial scales in the distribution. The outer
scale is that of the impact plume. The inner scale
is associated with localized, small scale convection
within the plume (Figure 2).
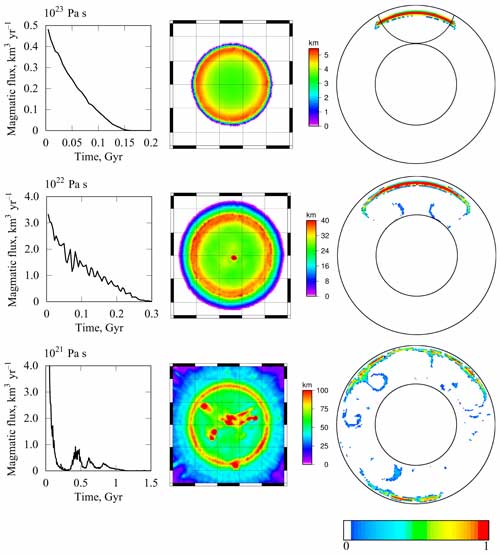
Figure 2. Magmatic evolution
of impact plume. Magmatic rate as a function of
time (left). Final spatial distribution of the
melt volume per unit surface area (center). The
grid line and frame intervals are 15¾. Final distribution
of mantle which has undergone partial melting
along a cross section passing through the impact
plume axis (right). Color (white through red)
indicates volume fraction of material which has
undergone partial melting and the solid line indicates
the radius of the initial intact melt region.
|
Discussion
Scaling relationships suggest
that for sufficiently large impacts the transient
crater is formed completely within the melt region
making retention of a final crater unlikely (Tonks
& Melosh, 1992). For smaller impacts,
elimination of initial crater structure formed
outside the melt region can occur during the processes
of isostatic adjustment and melt extrusion onto
the surface. The impact plume mechanism is capable
of producing additional magmatism associated with
the extended period of upwelling and decompression
melting. In this case, extensive magmatism would
not only erase transient crater structure but
might result in the development of a large igneous
province.
Mars Global Surveyor (MGS) topography
(Smith et al., 1999) plus material contained
within a depression due to Tharsis loading and
lithospheric flexure correspond to ~3 x 103
km3 of igneous material (Phillips
et al., 2001). The melt volume associated
with the initial impact can be compared to the
decompression melt volume produced by the upwelling
impact plume. For the low interior viscosity (1021
Pa s) case, the impact plume melt volume is of
the order of the impact-generated, retained melt
volume, ~109 km3 (Reese
et al.,
2004). It seems likely that, in this case,
the impact plume mechanism can contribute significantly
to the total extrusive melt volume. Large-scale
melting ceases by the end of the Noachian consistent
with timing of Tharsis formation. Perhaps a large
impactor, via extrusion of initial shock melt
and impact plume decompression melting, has the
potential to result in development of a large
volcanic construct such as Tharsis.
|
References
-
Agnor, C.B., R.M. Canup,
H.F. Levison, On the character and consequences
of large impacts in the late stage of terrestrial
planet formation, Icarus, 142,
219-237, 1999.
-
Albarede, F., J. Blichert-Toft,
J.D. Vervoort, J.D. Gleason, and M. Rosing,
Hf-Nd isotope evidence for a transient dynamic
regime in the early terrestrial mantle, Nature,
404, 488-490, 2000.
-
Banerdt, W.B., and M.P.
Golombek, Tectonics of the Tharsis region
of Mars: Insights from MGS topography and
gravity, Lunar Planet. Sci. Conf.,
31, #2038, 2000.
-
Breuer. D., D.A. Yuen,
T. Spohn, and S. Zhang, Three dimensional
models of Martian mantle convection with phase
transitions, Geophys. Res. Lett.,
25, 229-232, 1998.
-
Carr, M.H., Tectonism and
volcanism of the Tharsis region of Mars, J.
Geophys. Res., 79, 3943-3949,
1974.
-
Chambers, J.E., and G.W.
Wetherill, Making the terrestrial planets:
N-body integrations of planetary embryos in
three dimensions, Icarus, 136,
304-327, 1998.
-
Grasset, O., and E.M. Parmentier,
Thermal convection in a volumetrically heated,
infinite Prandtl number fluid with strongly
temperature dependent viscosity: Implications
for planetary evolution, J. Geophys. Res.,
103, 18171-18181, 1998.
-
Halliday, A.N., H. Wanke,
J.-L. Birck, and R.N. Clayton, The accretion,
composition, and early differentiation of
Mars, Earth, Moon, and Planets, 96,
197-230, 2001.
-
Harder, H., Mantle convection
and the dynamic geoid of Mars, Geophys.
Res. Lett., 27, 301-304,
2000.
-
Harder, H., and U.R. Christensen,
A one-plume model of Martian mantle convection,
Nature, 380, 507-509,
1996.
-
Harper, C.L., L.E. Nyquist,
B. Bensal, H. Wiesmann, and C.-Y. Shih, Rapid
accretion and early differentiation of Mars
indicated by 142Nd/144Nd in SNC meteorites,
Science, 267, 213-217,
1995.
-
Hartmann, W.K., Martian
surface and crust: Review and synthesis, Icarus,
19, 550-575, 1973.
-
Johnson, C.L., and R.J.
Phillips, Constraints on the evolution of
the Tharsis region of Mars, Lunar Planet.
Sci. Conf., 33, #1360,
2003.
-
Karato, S.-I., Does partial
melting reduce the creep strength of the upper
mantle?, Nature, 319,
8151-8176, 1986.
-
Karato, S.-I., and P. Wu,
Rheology of the upper mantle: A synthesis,
Science, 260, 771-778,
1993.
-
Kleine, T, K. Mezger, C.
Munker, H. Palme, and A. Bischoff, 182Hf –
182W isotope systematics of chondrites, eucrites
and Martian meteorites: Chrononlogy of core
formation and early diffentiation of Vesta
and Mars, Geochim. et Cosmochim. Acta,
68, 2935-2946, 2004.
-
Lee, D.C., and A.N. Halliday,
Core formation on Mars and differentiated
asteroids, Nature, 388,
854-857, 1997.
-
Lowry, A. R., and S. Zhong,
Surface versus internal loading of the Tharsis
rise, Mars, J. Geophys. Res., 108,
10.1029/2003JE002111, 2003.
-
Marty, B., and K. Marti,
Signatures of early differentiation of Mars,
Earth Planet. Sci. Lett., 196,
251-263, 2002.
-
McEwen, A.S., M.C. Malin,
M.H. Carr, and W.K. Hartmann, Voluminous volcanism
on early Mars revealed in Valles Marineris,
Nature, 397, 584-586,
1999.
-
Melosh, H.J., Giant impacts
and thermal state of the early Earth, in Origin
of the Earth, edited by H.E. Newsom and
J.H. Jones, Oxford Univ. Press, New York,
1990.
-
Nimmo, F., and D. Stevenson,
The influence of early plate tectonics on
the thermal evolution and magnetic field of
Mars, J. Geophys. Res., 105,
11969-11979, 2000.
-
Pierazzo E., A. M. Vickery,
and H. J. Melosh, A reevaluation of impact
melt production, Icarus, 127,
408-423, 1997.
-
Phillips, R.J., and E.R.
Ivins, Geophysical observations pertaining
to solid-state convection in the terrestrial
planets, Phys. Earth Planet. Inter.,
19, 107-148, 1979.
-
Phillips, R.J., M.T. Zuber,
S.C. Solomon, M.P. Golombek, B.M. Jakosky,
W.B. Banerdt, D.E. Smith, R.M.E. Williams,
B.M. Hynek, O. Aharonson, and S.A. Hauck,
Ancient Geodynamics and global scale hydrology
on Mars, Science, 291,
2587-2591, 2001.
-
Reese, C.C., V.S. Solomatov,
and L.-N. Moresi, Heat transport efficiency
for stagnant lid convection with dislocation
viscosity: Application to Mars and Venus,
J. Geophys. Res., 103,
13,643-13,657, 1998
-
Reese, C.C., V.S. Solomatov,
and J.R. Baumgardner, Survivial of impact
induced thermal anomalies in the Martian mantle,
J. Geophys. Res., 107,
10.1029/2000JE001474, 2002.
-
Reese,
C.C., V.S. Solomatov, J.R. Baumgardner, D.R.
Stegman, and A.V. Vezolainen, Magmatic evolution
of impact induced Martian mantle plumes and
the origin of Tharsis, J. Geophys. Res.,
109, E08009, doi:10.1029/2003JE002222,
2004.
-
Schubert, G., S.C. Solomon,
D.L. Turcotte, M.J. Drake, and N.H. Sleep,
Origin and thermal evolution of Mars, in Mars,
edited by H.H. Kieffer, B.M. Jakosky, C.W.
Snyder, and M.S. Matthews, pp. 147-183, Univ.
of Ariz. Press, Tucson, 1992.
-
Schultz, P.H., Impact basin
control of volcanic and tectonic provinces
on Mars, Lunar Planet. Sci. Conf.,
14, 728-729, 1984.
-
Schultz, P.H., and H. Glicken,
Impact crater and basin control of igneous
processes on Mars, J. Geophys. Res.,
84, 8033-8047, 1979.
-
Schultz, R.A., and H.V.
Frey, A new survey of large multiring impact
basins on Mars, J. Geophys. Res.,
95, 14175-14189, 1990.
-
Sleep, N.H., Martian plate
tectonics, J. Geophys. Res., 99, 5639-5655,
1994.
-
Smith, D.E., M.T. Zuber,
S.C. Solomon, R.J. Phillips, J.W. Head, J.B.
Garvin, W.B Banerdt, D.O. Muhleman, G.H. Pettengill,
G.A. Neumann, F.G. Lemoine, J.B. Abshire,
O. Aharonson, C.D. Brown, S.A. Hauck, A.B.
Ivanov, P.J. McGovern, H.J. Zwally, T.C. Duxbury,
The global topography of Mars and implications
for surface evolution, Science, 284,
1495-1503, 1999.
-
Solomatov, V.S., Fluid
dynamics of magma oceans, in Origin of
the Earth and Moon, edited by R. Canup
and K. Righter, Univ. Arizona Press, Tucson,
2000.
-
Solomatov, V.S., and L.-N.
Moresi, Time dependent stagnant lid convection
on the Earth and other terrestrial planets,
J. Geophys. Res., 105,
21795-21817, 2000.
-
Solomon, S.C., and J.W.
Head, Evolution of the Tharsis province of
Mars: The importance of heterogeneous lithospheric
thickness and volcanic construction, J.
Geophys. Res., 82, 9755-9774,
1982.
-
Spohn, T., Mantle differentiation
and thermal evolution of Mars, Mercury, and
Venus, Icarus, 90,
222-236, 1991.
-
Spohn, T., F. Sohl, and
D. Breuer, Mars, Astr. and Astrophys.
Rev., 8, 181-235, 1998.
-
Tajika, E., and S. Sasaki,
Magma generation on Mars constrained from
40Ar degassing model, J. Geophys. Res.,
101, 7543-7554, 1996.
-
Tonks, W.B., and H.J. Melosh,
Core formation by giant impacts, Icarus,
100, 326-346, 1992.
-
Tonks, W.B., and H.J. Melosh,
Magma ocean formation due to giant impacts,
J. Geophys. Res., 98,
5319-5333, 1993.
-
Trompert, R.A. and U. Hansen,
On the Rayleigh number dependence of convection
with a strongly temperature-dependent viscosity,
Phys. Fluids, 10,
351-360, 1998.
-
Turcotte, D.L., Thermal
evolution of Mars and Venus including irreversible
fractionation, Lunar Planet. Sci. Conf.,
20, 1138-1139, 1989.
-
Weidenschilling, S. J.,
D. Spaute, D. R. Davis, F. Marzari, and K.
Ohtsuki, Accretional evolution of a planetesimal
swarm. 2. The terrestrial zone, Icarus,
126, 429-455, 1997.
-
Weinstein, S.A., The effects
of a deep mantle endothermic phase change
on the structure of thermal convection in
silicate planets, J. Geophys. Res.,
100, 11,719-11,728, 1995.
-
Wetherill G.W., Occurrence
of giant impacts during the growth of the
terrestrial planets, Science, 228,
877-879, 1985.
-
Wetherill G.W., Formation
of the Earth, Annu. Rev. Earth Planet.
Sci., 18, 205-256, 1990.
-
Yoder, C. F., A. S. Konopliv,
D. N. Yuan, E. M. Standish, and W. M. Folkner,
Fluid core size of Mars from detection of
the Solar tide, Science, 300,
299-303, 2003.
-
Zaranek, S.E., and M.E.
Parmentier, Convective cooling of an initally
stably stratified fluid with temperature dependent
viscosity: Implications for the role of solid
state convection in planetary evolution, J.
Geophys. Res., 109,
B03409, doi:10.1029/2003JB002462, 2004.
-
Zhong, S., Effects of lithosphere
on the long wavelength gravity anomalies and
their implications for the formation of the
Tharsis rise on Mars, J. Geophys. Res.,
107, 10.1029/2000JE001589,
2002.
-
Zhong, S., and J. H. Roberts,
On the support of the Tharsis Rise on Mars,
Earth PLanet. Sci. Lett., 214,
1-9, 2003.
-
Zuber, M.T., S.C. Solomon,
R.J. Phillips, D.E. Smith, G.L. Tyler, O.
Aharonson, G. Balmino, W.B. Banerdt, J.W.
Head, C.L. Johnson, F.G. Lemoine, P.J. McGovern,
G.A. Neumann, D.D. Rowlands, S. Zhong, Internal
structure and early thermal evolution of Mars
from Mars Global Surveyor topography and gravity,
Science, 287, 1788-1793,
2000.
-
Zuber, M.T.,The crust and
mantle of Mars, Nature, 412,
220-227, 2001.
|
last updated November 29th,
2004 |
|
|
|