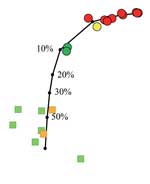 |
Trace Element and Isotopic Evidence for Recycled Lithosphere from Basalts from 48-53°E, Southwest Indian Ridge |
Jixin Wang1,2, Huaiyang Zhou1,*, Vincent J. M. Salters3, Henry J. B. Dick1,4, Jared J. Standish4,† & Conghui Wang1
1State Key Laboratory of Marine Geology, Tongji University, Shanghai 200092, China; wangjixin@qdio.ac.cn ; zhouhy@tongji.edu.cn ; hdick@protonmail.com ; 610739633@qq.com
2Center of Deep Sea Research, Institute of Oceanology, Chinese Academy of Sciences, Qingdao 266071, China
3National High Magnetic Field Laboratory and Department of Earth, Ocean and Atmospheric Science, Florida State University, Tallahassee, FL 32306, USA; salters@magnet.fsu.edu
4Department of Marine Geology and Geophysics, Woods Hole Oceanographic Institution, Woods Hole, MA 02543, USA; jeff.standish@gmail.com
*Corresponding author. Telephone: 86 13816928099. E-mail: zhouhy@tongji.edu.cn
†Present address: American Chemical Society, 1155 Sixteenth Street, N.W., Washington, DC 20036, USA.
This webpage is a summary of: Wang, J., Zhou, H., Salters, V.J.M., Dick, H.J.B., Standish, J.J. and Wang, C., 2020. Trace Element and Isotopic Evidence for Recycled Lithosphere from Basalts from 48 to 53°E, Southwest Indian Ridge. J. Pet., 61(10).
1. Overview
The Southwest Indian Ridge (SWIR) is a typical ultraslow spreading ridge, along which magmatic and amagmatic spreading modes alternate ubiquitously, thus resulting in large along-axis variations in magma production and crustal thickness. In the central part of the SWIR there are two strongly contrasting ridge segments (Figure 1). These are the magmatically robust 350-km-long eastern section of the 600-km-long Dragon Flag Supersegment (46°12′–52°18′E), called the Dragon Flag segment (48°25′-52°18′E), and the 80-km-long Dragon Bone amagmatic segment (52°22′-53°21′E). The Dragon Flag segment is generally overlain by a 3-10 km thick crust, probably some of the thickest crust anywhere along the SWIR (Niu et al., 2015). In contrast, the crust over the adjacent Dragon Bone segment is very thin and often missing (Zhou & Dick, 2013). Basaltic glasses recovered along the two adjacent segments show remarkable geochemical differences, triggering our interest in investigating why this is.
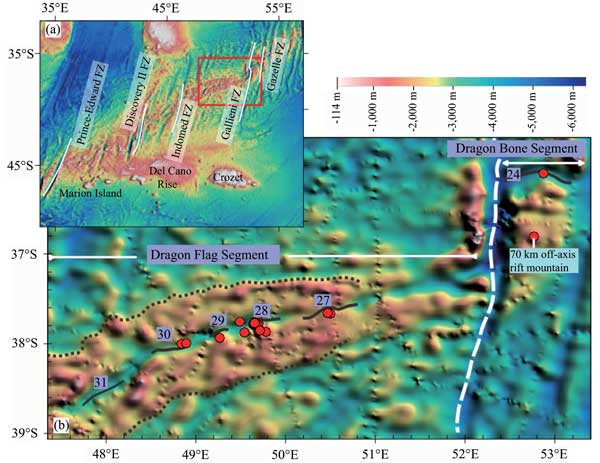
Figure 1. Bathymetric map of the central SWIR, the Dragon Flag Segment and the Dragon Bone Amagmatic Segment, with sample locations.
2. Geochemical Differences between the Dragon Bone and Dragon Flag basalts
The isotopic compositions of the Dragon Bone basalts are more enriched than average mid-ocean ridge basalt (MORB) (Gale et al., 2013) but their trace elements are close to average MORB (Figure 2). Conversely, the Dragon Flag basalts have isotopic compositions closer to average MORB but their trace element ratios are more extreme. That is to say, the extreme trace element ratios occur in samples whose isotopic characteristics are close to average MORB.
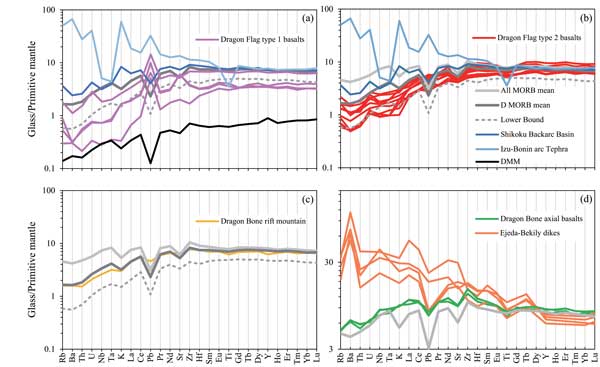
Figure 2. Primitive mantle normalized trace element patterns. The Dragon Flag basalts are sorted into two types due to the differences in trends of their trace elements. Click here or on Figure for enlargement.
3. Mechanisms that would affect the geochemical differences
Several factors can affect the geochemical evolution of the basalts erupted onto the seafloor:
- Mantle source variations;
- Partial melting processes;
- Post-melting processes, such as fractional crystallization.
The Dragon Bone basalts are clearly more enriched in isotopic composition than the adjacent Dragon Flag basalts (Figure 3), indicating that the two probably have different source compositions. But to what extent can the partial melting and post-melting processes affect their geochemical evolutions? Here we modeled the fractionation and partial melting effects based on their major and trace element compositions and found that neither post-melting processes nor partial melting from a single mantle source can explain the large difference of trace element ratios between the Dragon Bone and Dragon Flag basalts. Source composition variations could, however, largely account for the geochemical differences.
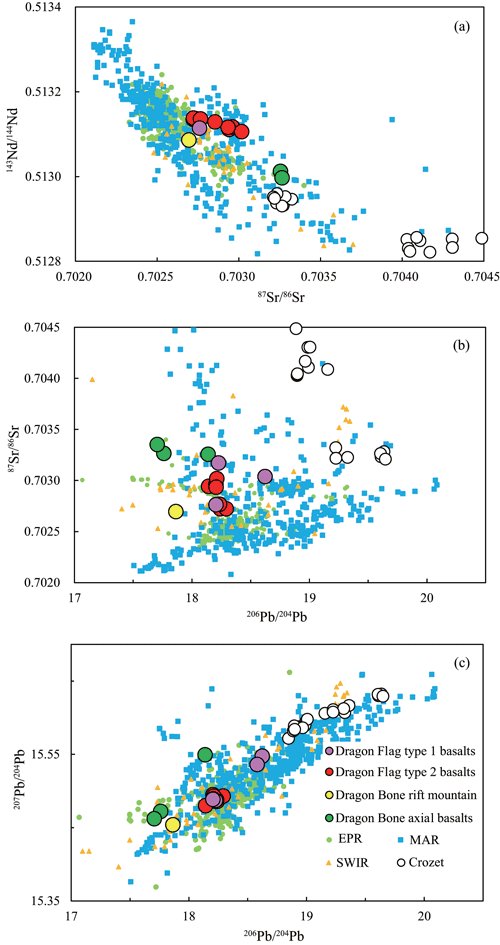
Figure 3. Isotopic distribution of the Dragon Flag and Dragon Bone basalts, compared with the Crozet hotspots, the EPR, the MAR and the SWIR.
4. Finding the source origins
Although the trace element ratios of the Dragon Flag and Dragon Bone basalts are within the range of global MORB, unique about these basalts is the good correlations between their trace elements and isotopic composition (Figure 4). The Dragon Bone axial basalts almost always lie at or near the extension of the Dragon Flag basalts, leading us to conclude that they share a similar mantle component. In addition, some of the Dragon Flag basalts have Th/U and La/Sm ratios down to 2.5 and 0.68 respectively, lower than the 2.9 and 0.87 of MORB source mantle (Salters & Stracke, 2004). Such low ratios imply that the shared mantle source which was mainly imprinted in the Dragon Flag basalts is more depleted than common normal MORB source.
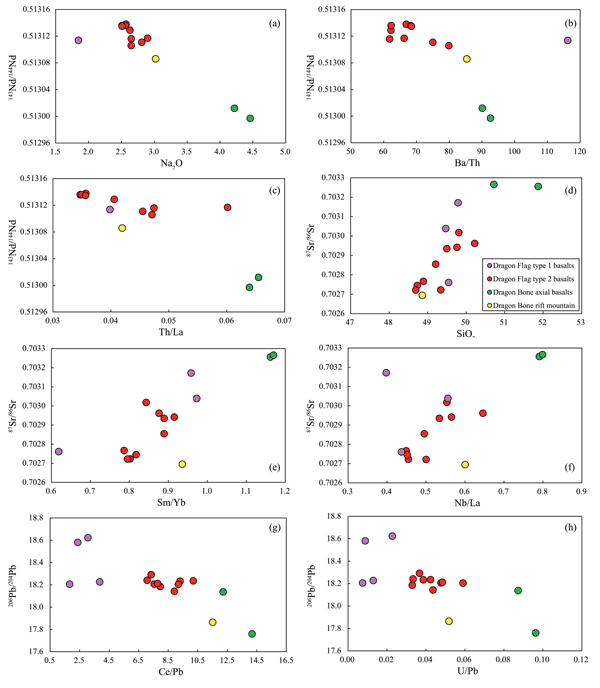
Figure 4. Variation of isotopic compositions with selected major elements and trace element ratios. Click here or on Figure for enlargement.
4.1 The Dragon Flag basalt source
4.1.1 Debate on the origin of the Dragon Flag basalt source
Mainly two scenarios have been proposed in the literature for the possible sources of the Dragon Flag basalts–influence by the Crozet hotspot and the involvement of subduction-modified sub-arc mantle. The first scenario was proposed by Sauter et al. (2009) to explain the high magma production at the Dragon Flag segment. Later on, Breton et al. (2013) found evidence for contamination by Crozet hotspot material based on Sr-Nd-Pb-He isotopes from the Crozet Archipelago. Yang et al. (2017) found isotopic evidence for the influence of the Crozet hotspot from Dragon Flag basalts only at 50.5°E on the SWIR. The second possibility was proposed by Li et al. (2017) as a result of observed higher H2O in Dragon Flag basalts compared with most N-MORB. Their high H2O contents and high H2O/Ce ratios are similar to those of back-arc basin basalt (BABB).
4.1.2 New evidence for the origin of the Dragon Flag basalt source
Our new trace element and isotopic compositions of Dragon Flag basalts do not support influence from the Crozet hotspot. As explained above, the Dragon Flag basalts were sourced from a mantle more depleted than common N-MORB source. Geochemically enriched hotspot-related materials are unlikely to generate such depleted melts, even after suffering a subsequent depletion beneatha mid-ocean ridge. By comparing 206Pb/204Pb–87Sr/86Sr data (Figure 3), it is clear that both west and east Crozet basalts cannot contribute significantly to the Dragon Flag basalts.
A sub-arc mantle origin is more likely. Trace elements of the Dragon Flag basalts have lower Zr/Sm, Th/U, Ce/Pb and higher La/Nb than average BABB (Gale et al., 2013) (Figure 5), indicating enrichment in fluid-mobile elements over immobile elements in the trace element pairs. These signatures are typical for fluid addition in an arc-mantle settings (Straub et al., 2009). Also, the high Ba/La at very low Th/Yb (Figure 6) in the Dragon Flag basalts also indicates slab-fluid addition (Elliott et al., 1997; Woodhead et al., 2001).
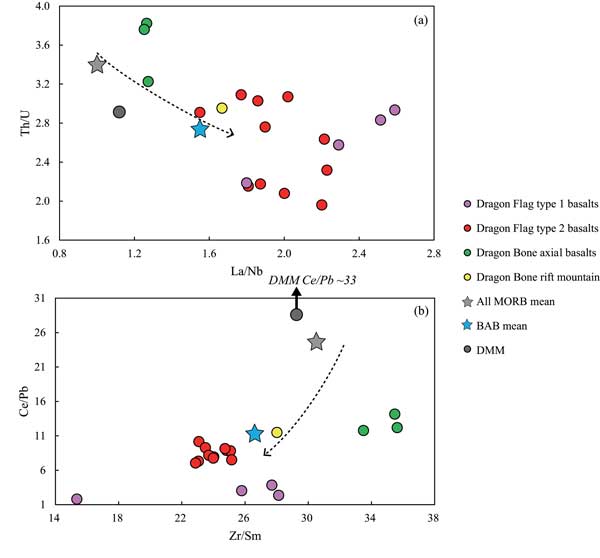
Figure 5. (a) Th/U vs La/Nb and (b) Ce/Pb vs Zr/Sm of the Dragon Flag basalts and the Dragon Bone basalts compared with average MORB, BABB and DMM values.
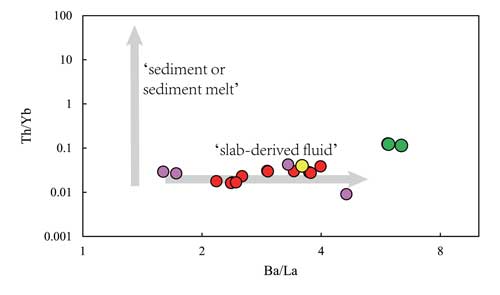
Figure 6. The relatively high Ba/La ratios of the Dragon Flag (type 1 and type 2) basalts, and the Dragon Bone axial and rift mountain basalts at very low Th/Yb indicating the involvement of slab-derived fluid.
Hydrous fluid-metasomatized mantle can melt to a greater extent under hydrous sub-arc conditions before its entrainment to beneath the mid-ocean ridge because fluid flux would lower its solidus temperature (e.g., Gaetani & Grove, 1998), thus producing a very refractory melting residue (e.g., Parkinson & Pearce, 1998; Gao et al., 2016; Wang et al., 2019). Such a refractory residue can generate more depleted melt during subsequent melting at a mid-ocean ridge and produce basalts more depleted than global average MORB.
We established a geochemical model to quantitatively constrain the amount of recycled material, the time of recycling and the time of sub-arc depletion. We found that the amount of slab fluid added to the sub-arc mantle cannot be more than 20%, the time of sudction should be 0.9 – 1.5 Ga, long before the assembly of Gondwana, and the depletion event of the subduction-modified sub-arc mantle should occur at 0.2 – 0.7 Ga, before opening of the SWIR (Figure 7).
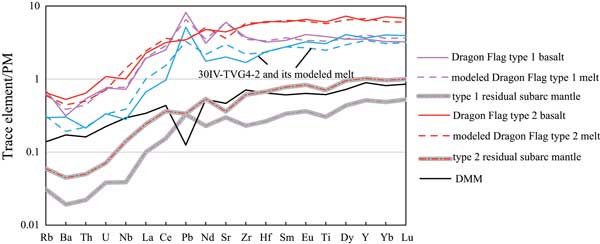
Figure 7. Primitive mantle normalized trace element patterns of the modeled melts (dashed lines) by two-stage depletion of a sub-arc mantle that was affected by slab–fluid addition, compared with the most primitive basalts in each type of basalt (continuous lines).
4.2 Dragon Bone axial basalt source
In geochemical diagrams, the Dragon Bone axial basalts almost always lie at or near the extension of the Dragon Flag basalt fields, leading us to conclude that the Dragon Bone axial basalts originated from a similarly depleted mantle source that imprinted in the Dragon Flag basalts. In addition to the Dragon Flag source as an endmember in the source of the Dragon Bone axial basalts, based on the similarity with the Ejeda-Bekily dikes and basalts from the 39-41°E SWIR (e.g., Mahoney et al., 1991; Dostal et al., 1992; Janney et al., 2005) a component of delaminated subcontinental lithospheric mantle is proposed as another endmember (Figure 8).
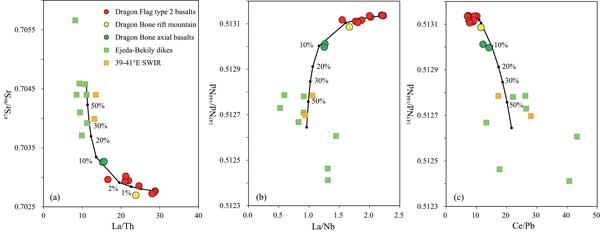
Figure 8. Mixing of the isotopically most depleted Dragon Flag basalt with the average of the Ejeda–Bekily dikes on the 39–41°E SWIR.
5. Geologic history of the central SWIR
The major factor that determines the geochemical differences between the Dragon Flag and the Dragon Bone basalts is the difference in their mantle source origins. The recycled residual sub-arc mantle that feeds the Dragon Flag basalts, together with the subcontinental lithospheric mantle source component, could be related to either the breakup of Gondwana or the formation and accretion of Neoproterozoic island arc terranes during the collapse of the Mozambique Ocean. Whichever is correct, it is now present beneath the Southwest Indian Ridge.
References
- Breton, T., Nauret, F., Pichat, S., Moine, B., Moreira, M., Rose- Koga, E. F., Auclair, D., Bosq, C. & Wavrant, L.-M. (2013). Geochemical heterogeneities within the Crozet hotspot. Earth and Planetary Science Letters 376, 126–136.
- Dostal, J., Dupuy, C., Nicollet, C. & Cantagrel, J. M. (1992). Geochemistry and petrogenesis of upper Cretaceous basaltic rocks from southern Malagasy. Chemical Geology 97, 199–218.
- Elliott, T., Plank, T., Zindler, A., White, W. & Bourdon, B. (1997). Element transport from slab to volcanic front at the Mariana arc. Journal of Geophysical Research 102, 14991–15019.
- Gaetani, G. A. & Grove, T. L. (1998). The influence of water on melting of mantle peridotite. Contributions to Mineralogy and Petrology 131, 323–346.
- Gale, A., Dalton, C. A., Langmuir, C. H., Su, Y. & Schilling, J. G. (2013). The mean composition of ocean ridge basalts. Geochemistry, Geophysics, Geosystems 14, 489–518.
- Gao, C., Dick, H. J., Liu, Y. & Zhou, H. (2016). Melt extraction and mantle source at a Southwest Indian Ridge Dragon Bone amagmatic segment on the Marion Rise. Lithos 246–247, 48–60.
- Janney, P., Le Roex, A. & Carlson, R. (2005). Hafnium isotope and trace element constraints on the nature of mantle heterogeneity beneath the central Southwest Indian Ridge (13°E 47°E). Journal of Petrology 46, 2427–2464.
- Li, W., Jin, Z., Li, H. & Tao, C. (2017). High water content in primitive mid-ocean ridge basalt from Southwest Indian Ridge (51.5°E): implications for recycled hydrous component in the mantle. Journal of Earth Science 28, 411–421.
- Mahoney, J. J., Nicollet, C. & Dupuy, C. (1991). Madagascar basalts: tracking oceanic and continental sources. Earth and Planetary Science Letters 104, 350–363.
- Niu, X., Ruan, A., Li, J., Minshull, T. A., Sauter, D., Wu, Z., Qiu, X., Zhao, M., Chen, Y. J. & Singh, S. (2015). Along-axis variation in crustal thickness at the ultraslow spreading Southwest Indian Ridge (50°E) from a wide-angle seismic experiment. Geochemistry, Geophysics, Geosystems 16, 468–485.
- Parkinson, I. J. & Pearce, J. A. (1998). Peridotites from the Izu–Bonin–Mariana forearc (ODP Leg 125): evidence for mantle melting and melt–mantle interaction in a supra-subduction zone setting. Journal of Petrology 39, 1577–1618.
- Salters, J. M. V. & Stracke, A. (2004). Composition of the depleted mantle. Geochemistry Geophysics Geosystems 5, Q05004, doi:10.1029/2003GC000597.
- Sauter, D., Cannat, M., Meyzen, C., Bezos, A., Patriat, P., Humler, E. & Debayle, E. (2009). Propagation of a melting anomaly along the ultraslow Southwest Indian Ridge between 46°E and 52°20′E: interaction with the Crozet hotspot? Geophysical Journal International 179, 687–699.
- Straub, S. M., Goldstein, S. L., Class, C. & Schmidt, A. (2009). Mid-ocean-ridge basalt of Indian type in the northwest Pacific Ocean basin. Nature Geoscience 2, 286–289.
- Wang, J., Zhou, H., Salters, V., Liu, Y., Sachi-Kocher, A. & Dick, H. (2019). Mantle melting variation and refertilization beneath the Dragon Bone amagmatic segment (53°E SWIR): major and trace element compositions of peridotites at ridge flanks. Lithos 324–325, 325–339.
- Woodhead, J. D., Hergt, J. M., Davidson, J. P. & Eggins, S. M. (2001). Hafnium isotope evidence for ‘conservative’ element mobility during subduction zone processes. Earth and Planetary Science Letters 192, 331–346.
- Yang, A. Y., Zhao, T.-P., Zhou, M.-F. & Deng, X.-G. (2017). Isotopically enriched N-MORB: a new geochemical signature of off-axis plume-ridge interaction—a case study at 50°28′E, Southwest Indian Ridge. Journal of Geophysical Research: Solid Earth 122, 191–213.
- Zhou, H. & Dick, H. J. (2013). Thin crust as evidence for depleted mantle supporting the Marion Rise. Nature 494, 195–201.
last updated 14th May, 2021 |