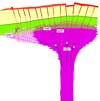 |
Continental
Flood Basalts and Mantle Plumes:
a Case Study of the Northern Ethiopian Plateau |
L. Beccaluva1*,
G. Bianchini1,2, C.
Natali1 & F. Siena1
1Dipartimento
di Scienze della Terra, Università di
Ferrara, Italy; bcc@unife.it, bncglc@unife.it, ntlcld@unife.it, snr@unife.it
2School of Geology, Geography and the Environment,
Kingston University, Kingston upon Thames,UK
Corresponding author: Luigi
Beccaluva
This webpage is an abridged version of the paper: Beccaluva,
L., G. Bianchini, C. Natali, and F. Siena, Continental
Flood Basalts and Mantle Plumes: a Case Study of
the Northern Ethiopian Plateau, J. Petrology, 50,
1377-1403; doi:10.1093/petrology/egp024
Click here for Discussion of this page
Introduction
There has been renewed scrutiny of
mantle plumes in the last decade. Many controversial
hypotheses have been proposed regarding the depth of
plume provenance, triggering mechanisms, shape and
size, relationships with hotspots, large igneous provinces
(LIPs), rift volcanism, and the very existence
of plumes has been questioned (Ernst & Buchan,
2001; Foulger
et al., 2005; Beccaluva et al., 2007b; Foulger & Jurdy,
2007). The East African rift system is of
particular interest as large Continental Flood Basalts
(CFB) were erupted during the Tertiary associated with
regional uplift (Ethiopian and Kenyan domes), and they
have been attributed to one or two distinct mantle
plumes (Ebinger & Sleep,
1998; Rogers et al., 2000; Yirgu et al.,
2006). The Early Oligocene Northern Ethiopia-Yemen
volcanic province comprises the entire range of CFB
magmas, from Low-Ti to High-Ti and very High-Ti basalts
and picrites, erupted in a well-defined space-time
interval, and overlain by younger alkaline basalts
with peridotite xenoliths. These provide direct evidence
of the nature of the mantle beneath the plateau. This
CFB province corresponds to the region where Afro-Arabia
continental break-up took place with the formation
of the Red Sea-Gulf of Aden-East African rift system
centred on the Afar triple junction, where a possible deep
mantle plume has been suggested on the basis of geophysical
data (Hofmann et al., 1997; Davaille et
al., 2005).
The Northern Ethiopian plateau (Figure
1) mainly consists of tholeiitic to transitional basaltic
lavas (Mohr & Zanettin,
1988), which were erupted at ~ 30 Ma in a period of
1 Ma or less (Hofmann et al., 1997). The
basalts cover an area of ~210,000 km2 with
a lava pile up to 2000 m thick in the central-eastern
part, thinning to less than 500 m toward the northern
and southern boundaries. On the eastern margin, neighbouring
the Afar escarpment, rhyolitic volcanic rocks characterizing
the upper part of the sequence have been interpreted
as the differentiated products of basaltic magmas that
mark the start of continental rifting (Ayalew et
al., 2006). Similar nearly coeval CFB volcanism
is recorded in the Yemen conjugate margin covering
an area of ~80,000 km2 (Baker et al.,
1996; Ukstins
et al., 2002). Plateau volcanism in Northern
Ethiopia was preceded by uplift (at least since the
Late Eocene, based on stratigraphical data: Bosellini
et al.,
1987) of the underlying basement by up to 1-2 km (Sengör,
2001). After the CFB emplacement, the area was affected
by a further ~2 km uplift, as a result of isostatic
and tectonic processes (Gani et al., 2007).
Dyke swarms, that most probably fed CFB fissure eruptions
(Mège & Korme, 2004; Figure 1),
are subparallel to sea-floor spreading axes in the
Gulf of Aden and Red Sea, suggesting multiple rifting
and spreading processes radiating from the still active
Afar zone (e.g., the volcano-seismic event
of 2005; Ayele et al., 2007).
Figure 1. Sketch map of the Oligocene
continental flood basalts (CFBs) of the Northern
Ethiopia and the Yemen conjugate margins, based on
the geological map by Merla et al. (1973),
NASA STRM images, data from Baker et al. (1996),
Pik et al. (1998), Kieffer et al. (2004)
and this work. LT, Low-Ti tholeiitic basalts; HT1,
High-Ti tholeiitic basalts; HT2, very High-Ti transitional
basalts and picrites. Dyke swarms after Mége & Korme
(2004); locations of mantle xenoliths hosted in Quaternary
alkaline volcanic rocks in the plateau area are also
indicated. Inset: regional geodynamic sketch map
with plate motions and velocities after Bellahsen
et al. (2003).
Geographical information system (GIS)-based
digitization of the 1:2,000,000 geological map of the
region (Merla et al., 1973), carried out as
part of this study, permitted the original volume of
the flood basalts in the Northern Ethiopian Plateau
to be estimated as 250,000 km3, implying
an eruption rate of the order of 0.2-0.3 km3/year,
which is comparable with that of other CFB provinces
(Farmer, 2003). The plateau basalts are overlain
by huge shield volcanoes mainly composed of alkaline
lavas (Mt. Choke and Gugugftu, 22 Ma; Mt. Guna, 10.7
Ma; Kieffer et al., 2004). In the southwestern
part of the plateau highly alkaline lavas were erupted
from a number of Quaternary volcanic centres. Basanite
lavas from two of these volcanoes near Injibara and
west of Nekemte (Dedessa River) carry abundant mantle
xenoliths, which have also been considered in this
study.
The new major and trace-element analyses
of the Northern Ethiopian plateau basalts confirm the
existence of three main magma types spatially zoned
according to their TiO2 content (Pik
et al., 1998) and
allow a more precise definition of their zonal arrangement
(Figure 1). Low-Ti tholeiites (LT), in the NW, are
quantitatively predominant (150,000 km3).
High-Ti lavas (HT1) predominate southeastward. Ultra-titaniferous
transitional basalts and picrites (HT2) are concentrated
in the Lalibela area, closer to the centre of the Afar
triangle. In both alkali vs silica (Figure
2) and TiO2 vs MgO (Figure 3) diagrams
distinctive compositional features of the three magma
types can be observed. LT basalts mostly plot in the
subalkaline field, showing the widest differentiation
range with MgO 3-12 wt %, coupled with the lowest TiO2 contents
(1.0-3.5 wt %). The HT1 group is mainly composed of
tholeiitic basalts with MgO 4-9 wt % and TiO2 2.5-4.8
wt %. HT2 transitional basalts and picrites cluster
around the alkaline-subalkaline boundary with MgO 5-12
wt % and TiO2 3.5-5.9 wt % for basalts,
and MgO 13-16 wt % and TiO2 2.6-4.5 wt %
for picrites. Moreover, there is a broad correlation
between TiO2 and
Fe2O3 tot, the latter increasing
from 11-12 wt % in the least differentiated LT to 14-15
wt % in the HT lavas (not shown).
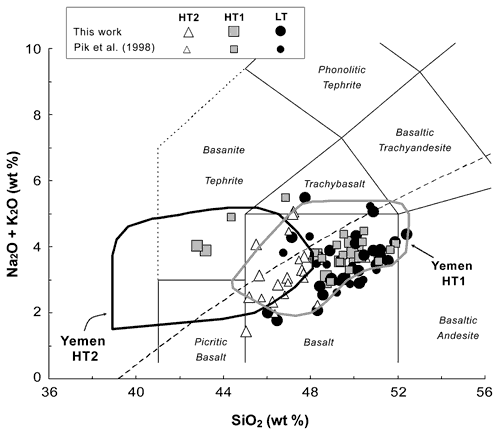
Figure 2. Total alkali-silica classification
diagram (Le Bas et al., 1992) for the Northern Ethiopia
CFBs (data from this work and Pik et al., 1998).
For comparison, data from coeval Yemen CFBs have
also been plotted (from Baker et al., 1996; and authors’ unpublished
data). LT, Low-Ti tholeiites; HT1, High-Ti tholeiites;
HT2, very High-Ti transitional basalts and picrites.
The dashed line dividing alkalic and subalkalic series
is after Irvine & Baragar (1971).
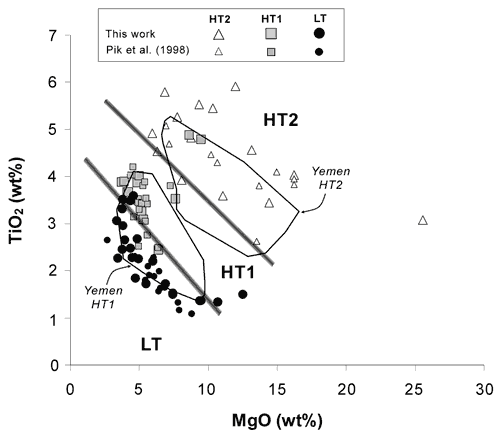
Figure 3. TiO2 vs MgO (wt %) for
the Northern Ethiopian CFBs (data from this study
and Pik et al., 1998). For comparison data from coeval
Yemeni CFBs have also been plotted (from Baker et
al., 1996; and authors’ unpublished data).
LT, Low-Ti tholeiites; HT1, High-Ti tholeiites; HT2,
very High-Ti transitional basalts and picrites. Empirical
intergroup boundaries are drawn to minimize misclassified
samples.
The decreasing silica saturation
from LT to HT2 is reflected in the parallel decrease
in normative hypersthene from 12-23% in LT and HT1
tholeiites to < 10% in many HT2 transitional
basalts and picrites. CFBs from the coeval Yemen
plateau (Manetti et al., 1991; Baker
et al., 1996; author’s unpublished data)
are classified as HT1 and HT2, and show striking
compositional similarities with the Northern Ethiopian
High-Ti lavas facing the Afar triangle (Figures 2
and 3). This further supports the hypothesis that,
before the opening of the Afar-Red Sea system, these
volcanic districts would have been part of the same
magmatic province extending ~700 km in an east-west
direction. A regional comparison shows that the LT
and HT1 lavas from the Ethiopia-Yemen province show
close similarities to other Low- and High-Ti CFB
provinces (e.g., Paranà, Piccirillo & Melfi,
1988; Deccan, Melluso et al., 1995). On
the other hand, the distinctive compositions of the
HT2 ultra-titaniferous basalts and picrites seem
to be only partially comparable with those of some
picrites from the Karoo igneous province (Sweeney
et al., 1991; Cawthorn & Biggar,
1993; Ellam, 2006) and the Siberian Traps
(meimechites: Campbell et al., 1992).
Petrogenetic modelling
Different approaches
based on major elements were used to develop a comprehensive
petrogenetic model for the least differentiated CFBs
from the three groups, which all include (with the
exception of HT1) samples with Mg# 0.68-0.72, that
is, in equilibrium with mantle sources containing olivine
Fo > 88 (Herzberg & O’Hara,
2002; Green & Falloon, 2005). Most of
the Northern Ethiopian CFBs have lower Mg# and only
a few HT2 picrites (non-olivine-cumulative) reach
the threshold of Mg# 0.72 that was considered by Niu & O’Hara (2008)
as the required value for true primary magmas. However,
the relatively low Mg# values of CFBs from Northern
Ethiopia are, at least in part, related to the distinctive
Fe enrichment that appears to be a primary feature
of these magmas. Therefore, model calculations, reported
below, have been carried out on a large dataset (also
including analyses by Pik et al., 1998)
for basalts with MgO > 6%, which represents the compositional
limit for olivine to be considered the main phase
controlling the liquid line of descent (Herzberg & Asimow,
2008).
The empirical model of Niu & Batiza (1991)
was first applied to estimate the degree of melting
(F), and the initial (P0) and final (Pf) melting pressure
of the Northern Ethiopian basalts, assuming that they
formed along a melting column from mantle sources under
decompression conditions. Basalt compositions out of
the range of the experimental data on which the model
was based were discarded and analyses with MgO < 8%
were normalized to MgO = 8% by adding an appropriate
amount of olivine Fo87, which represents
the most magnesian phenocryst composition observed
in the Northern Ethiopian basalts. These data were
integrated with the pressure (PA) and temperature (T)
of melt segregation calculated using the algorithm
of Albaréde (1992).
For picrites, which fall outside the composition range
investigated by Niu & Batiza (1991), only
the Albaréde thermobarometric approach was used,
and F=25-30% was assumed in accordance with experimental
petrology data (Green & Falloon, 2005,
and references therein).
Results are reported in the
P-T petrogenetic grid of Figure 4. Temperatures of
basalt segregation range from 1400°C for HT to
1200°C for LT. In picrites,
higher segregation temperatures (up to 1500°C)
are recorded in accordance with both the exceedingly
high Ni content (1100-650 ppm in bulk-rocks) and the
high Cr content of their olivine phenocrysts (up to
1000 ppm), which conform with plume-derived superheated
magmas (Campbell, 2001).
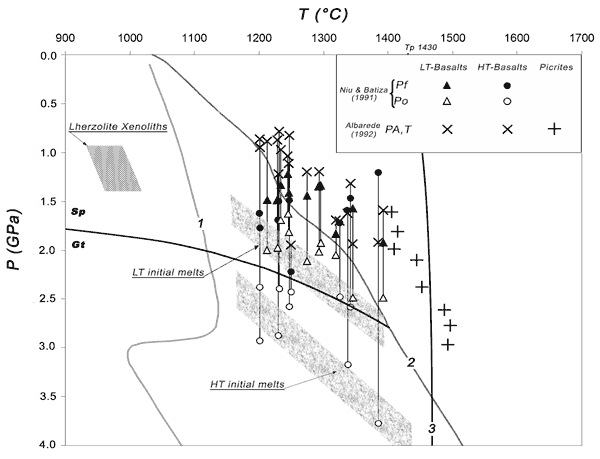
Figure 4. Pressure (P)-temperature
(T) petrogenetic grid depicting the melting conditions
of Northern Ethiopian CFBs based on the model proposed
by Niu & Batiza (1991) (P0 and Pf are initial
and final pressures of melt formation) and Albarede
(1992) (PA and T are pressure and temperature of
melt segregation). Results from this study and Pik
et al. (1998). Volatile (H-O-C)-bearing (1) and dry
(2) lherzolite solidi, spinel (sp) and garnet (gt)
stability fields, and adiabat (3) for mantle potential
temperature Tp 1430°C are after Green & Falloon
(2005). Shaded fields refer to the P-T equilibration
conditions of lherzolite mantle xenoliths from Northern
Ethiopia (this work and Conticelli et al., 1999),
HT and LT initial melts. (See text for further explanation.)
Further temperature estimates for
picrites have been obtained using olivine-liquid thermometers
according to Ford et al. (1983) and Herzberg & O’Hara (2002),
giving temperatures of ~ 1400°C (in equilibrium
with Fo90 olivine) and 1360-1365°C (in
equilibrium with Fo88 olivine) for samples LAL6 and
LAL9, respectively. As expected, these olivine liquidus
temperatures are systematically lower (by some 50-100°C)
than those calculated for magma segregation from the
mantle sources. This allows us to conclude that, even
considering the possible H2O effect in lowering
the liquidus temperature (Falloon et al.,
2007), the generation conditions of the hottest (picritic)
magmas has to be ≥ 1350°C.
These
results emphasize that the zonal arrangement of Northern
Ethiopian CFBs from LT in the west to HT2 in the
east broadly corresponds to a parallel increase in
magma temperature. Moreover, the temperature difference
between the hottest Ethiopian CFBs and the ambient
mantle, represented by mantle xenoliths from the area
(Figure 4), is at least > 300°C, which
is compatible with the thermal anomaly induced by a
deep mantle plume (Farnetani & Richards,
1994; Campbell,
2007). The geothermal regime seems to vary from nearly
adiabatic (potential temperature Tp = 1430°C),
with generation of the hottest picritic magmas over
a pressure interval of 1.6-3.0 GPa, to non adiabatic,
where LT magmas were formed. This implies that the
mantle region that underwent partial melting had its
deepest and hottest part centred in the east, close
to the Afar triple junction, where a deep plume has
been proposed on the basis of seismic tomography (Davaille
et al., 2005). This is consistent with the
petrological model proposed by Herzberg & O’Hara (2002)
for plume-associated picritic magmas.
The interpolation
of the P0 values of the initial melts for the LT and
HT magmas defines two distinct near-solidus mantle
arrays located between the dry and the volatile bearing
peridotite solidi. The HT initial melting array is
closer to the H-O-C-bearing lherzolite solidus, suggesting
a more volatile-enriched nature for their mantle sources,
as also indicated by the significant presence of hydrous
phases in some HT basalts.
Major element mass-balance
calculations between the basalts and the lherzolite
xenoliths were also carried out to constrain
further the composition of the mantle sources, using
F estimates obtained from the model above. The modal
mineral composition of the mantle sources, as well
as the melting proportions, were defined by iterative
least-squares calculations based on the major-element
compositions of the constituent minerals plus additional
mantle phases such as amphibole. Phlogopite, garnet
and ilmenite were generally not present in the studied
lherzolite xenoliths, but required by computation.
A summary of the modelling results is given in Table
10 of Beccaluva
et al. (2009),
which relates each magma type with the source mineralogy,
melting proportions and degree, and the P-T conditions
of melting. This indicates the following features.
-
Chemical components corresponding
to amphibole ± phlogopite
are always required in the magma source, varying
from 7% for LT tholeiites to 10 -12% for HT basalts
and picrites, and are the predominant melting phases.
These phases, or another chemically equivalent
mineral assemblage stable at the relevant P-T
conditions, are considered to be related to metasomatizing
agents interacting with the original mantle parageneses,
and confirm the hydrated nature of the mantle solidus
hypothesized in Figure 4.
-
Titanium-rich minerals (e.g., ilmenite, rutile, armalcolite) are additional metasomatic
phases required in the mantle sources of the ultra-titaniferous
(HT2) magmas.
-
The presence of negative olivine in the melting
proportions of the subalkaline melts, although decreasing
from LT to HT1 tholeiites, reflects a significant incongruent
melting of orthopyroxene in the source of the tholeiitic
magmas (Beccaluva et al., 1998).
-
The significant presence of garnet required in
both the mantle source and the melting proportions
of the HT magmas confirms P0 estimates of their initial
melting conditions in the garnet lherzolite stability
field (Figure 4).
Further petrogenetic constraints are
provided by the distribution of those incompatible
trace elements that are widely recognized as indicators
of OIB mantle sources in hotspot or plume regions.
To evaluate quantitatively the incompatible-element
distribution in the magma sources, batch melting modelling
was carried out based on the basalt compositions, using
the melting parameters defined in the previous section.
Results show that to generate the entire range of Ethiopian
CFB magmas, from LT to HT, the calculated mantle sources
(SLT bas, SHT1 bas, SHT2 picr; Figure 5) should be
of the order of 2-15 times richer in incompatible
elements with respect to those of the most fertile
Northern Ethiopian mantle xenoliths. Significantly,
these source compositions satisfactorily match those
(S1, S2, S3; Figure 5) resulting from lherzolite xenoliths
GOJ26 and GOJ40A with the addition of an amount of
metasomatic amphibole plus Ti phases approaching that
required by the major element mass-balance calculations
(Table 1). The lherzolite xenoliths have flat mantle
normalized trace element patterns (0.7-1.0 x PM), showing
little sign of incompatible element enrichment, in
contrast to other xenolith occurrences in southern
Ethiopia (Bedini et al., 1997)
and along the Saharan Belt (Hoggar, Algeria, Beccaluva
et al., 2007a; Gharyan, Libya, Beccaluva et
al., 2008).
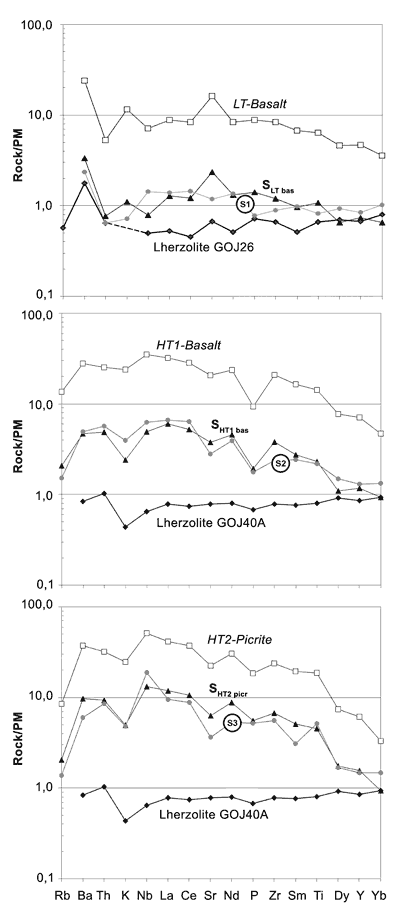
Figure 5. Primitive Mantle (PM)-normalized
(Sun & McDonough, 1989) incompatible-element
patterns of Northern Ethiopian LT and HT magmas and
their inferred mantle sources. SLT bas, SHT1 bas
and SHT2 picr refer to source compositions calculated
by batch melting for LT basalt SIM15, HT1 basalt
BLN4 and HT2 picrite LAL6, using partition coefficients
(Kd) from the GERM website (http://earthref.org/GERM)
and melting parameters as defined in Table 10 of Beccaluva
et al. (2009). These theoretical compositions compare
favourably with those of Ethiopian mantle xenoliths
GOJ26 and GOJ40A (Table 1 of Beccaluva
et al., 2009)
with the addition of appropriate metasomatic phases:
S1=lherzolite GOJ26 (ol 61, opx 22, cpx 14, sp 3) +
3% amphibole (from Zabargad lherzolites; Brooker et
al., 2004); S2=lherzolite GOJ40A (ol 60, opx 22, cpx
15, sp 3) + 9% amphibole (from Kerguelen mantle xenoliths;
Moine et al., 2001); S3=lherzolite GOJ40A + 12% amphibole
and 1% ilmenite (from Kerguelen mantle xenoliths; Grégoire
et al., 2000; Moine et al., 2001).
The consistency of the major- and
trace-element modelling suggests that the Northern
Ethiopian CFBs were generated from lherzolite mantle
sources variably metasomatized by alkali-silicate hydrous
melts enriched in high field strength elements (HFSE;
e.g., Ti, Nb, Zr), low field strength elements
(LFSE; e.g., Ba and Th), and LREE. These metasomatizing
agents show compositional similarities to those recorded
in mantle xenoliths from the Kerguelen Islands, where
both amphibole and Ti-rich metasomatic phases (including
rutile, ilmenite and armalcolite) have been reported
(Grégoire
et al.,
2000).
The interpretation of this metasomatic
enrichment is not straightforward, being related either
to a contribution from the Earth’s metallic core
(Humayun et
al., 2004) or to the involvement of eclogitic
materials recycled in the mantle (Sobolev et al.,
2007). However, the distinctive Ti enrichment observed
in the Northern Ethiopian CFBs implies that the metasomatizing
agents were enriched in this element, favouring an
origin from mantle sources that included Ti-rich eclogitic
materials. These materials most probably result from
the recycling of Ti-rich mid-ocean ridge basalt (MORB)
protoliths in the mantle via ancient subduction.
The
origin of the volatile components in the metasomatizing
agents is a matter of considerable debate. The helium
isotopic composition of Ethiopian CFBs shows Ra up
to 20 for some High-Ti magmas, suggesting an origin
from the undegassed lower mantle (Pik et al.,
2006) or even from the D" layer at the core -mantle
boundary (Tolstikhin & Hofmann, 2005).
Analogous considerations are valid for the relatively
high H2O contents in the HT magmas. From
the above it is evident that the inferred metasomatic
agents could have integrated various mantle geochemical
components with different provenance and mobility.
These components probably pooled by scavenging and
mixing of heterogeneous mantle materials during plume
ascent (Farnetani
et al., 2002).
Conclusions
The spatial
distribution of the various magma types, together with
their petrogenetic P-T-X constraints, are used to define
the shape, size and location of the melting region
in the underlying mantle where the magmas were generated.
Our results suggest an onion-like melting region (Figure
6), most probably representing the head of an impinging
deep mantle plume that induced a dramatic thermal anomaly,
lithospheric bulging or faulting, and the Early Oligocene
CFB volcanism in Northern Ethiopia-Yemen. The petrological
model presented here fits the first-order requirements
for a deep mantle plume hypothesis as predicted by
laboratory and numerical modelling (Farnetani & Richards,
1994; Campbell,
2007); that is, shape, dimensions, temperature excess
(ΔT > 300°C), lithosphere bulging, and
CFB timing.
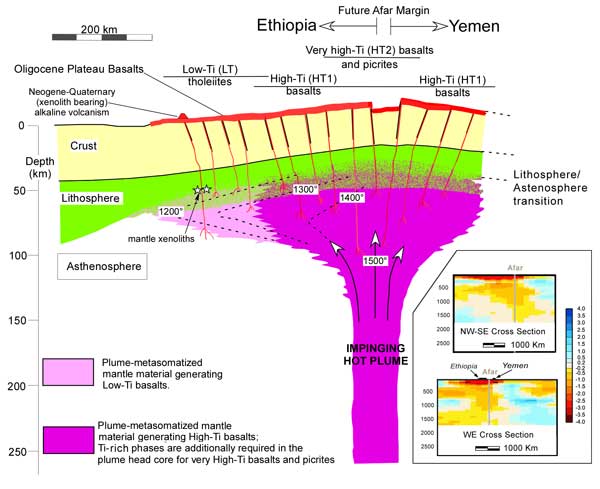
Figure 6. Schematic illustration
showing the Afar plume impinging on the Afro-Arabian
lithosphere and the generation of Oligocene Northern
Ethiopia-Yemen CFBs from a thermally and compositionally
zoned plume head. Mantle sources are affected by
decreasing metasomatic effects from the hottest core
of the plume head to the cooler outer zones. Metasomatic
agents are envisaged as alkali-silicate melts enriched
in multiple geochemical components (Ti, HFSE, LFSE,
LREE, H2O, noble gases, etc.) rising along
the plume axial zone. The hypothesized provenance depth
of mantle xenoliths entrained in Neogene-Quaternary
alkaline volcanic rocks is also indicated. The inset
shows mantle tomographic cross-sections beneath Afar,
based on models for shear-wave velocity variations
(Davaille et al., 2005). Low velocities are attributable
to the presence of less dense or hotter material
from a rising plume. Click here or
on figure for enlargement.
In this scenario multiple geochemical
components, pooled and upwelled along the plume stem,
effectively accumulated and spread laterally in the
plume head. The intensity of the metasomatism decreases
from the plume axial zone towards the periphery, as
reflected by the zonal arrangement of the erupted magmas,
which requires progressively less metasomatized mantle
sources in a westward direction. CFB generation in
Northern Ethiopia and the conjugate Yemen margin was
favoured by several factors:
- lowered solidus temperatures of plume metasomatized
mantle sources;
- heat transfer by the plume buoyancy flux that raised
the regional geotherm;
- decompression of the upwelling mantle.
As illustrated in Figure 6, these
factors can satisfactorily account for the generation,
at comparable melting degrees, of LT tholeiites from
the outer (westwards) comparatively less metasomatized
mantle sources and of HT1 basalts from the more metasomatized
mantle domains closer to the plume axis (eastwards).
Ultra-titaniferous HT2 transitional basalts and picrites
were generated in the innermost (hottest) part of the
Afar plume from highly metasomatized mantle domains,
which probably underwent nearly adiabatic decompression
(in the range 3.0 -1.6 GPa) starting from the garnet-peridotite
stability field.
Paleogeographical restoration of the
Yemen CFBs, nearly exclusively represented by HT magmas,
fits perfectly with the analogous HT lavas of the Northern
Ethiopian plateau, and suggests an asymmetric shape
and extension of the underlying plume head (Figure
6), as indicated by available seismic tomography data
(Davaille et al., 2005). The distinctive composition
and zonal arrangement of the Northern Ethiopia-Yemen
CFBs centred on the Afar triple junction further supports
a relationship with a mantle plume independent from
the Kenyan plume (Rogers et al., 2000). The
available Sr-Nd-Pb isotope data show a common isotopic
signature for CFBs from the Northern Ethiopia and Yemen
plateaux (Afar plume signature) and a distinct composition
with respect to the Southern Ethiopian and Kenyan lavas
(Rogers et al., 2000; Furman et al.,
2006; Rogers, 2006). Further evidence for
a distinct Afar plume is provided by recent shear-wave
velocity data and relative stratification of anisotropy
in the Afar region (Sicilia et al., 2008).
The geodynamic evolution of the region
after the CFB activity indicates that the African plate
was relatively stable with respect to the Afar plume,
with extension mainly focused along the Red Sea-Gulf
of Aden spreading system during Neogene-Quaternary
times. This implies that, while the African plate was
locked against the Alpine orogenic systems along the
Mediterranean and Bitlis collisional margins, the Arabian
plate could migrate northeastward, pulled by the active
Makran subduction, thus favouring the current oceanic
opening along the Red Sea-Afar-Gulf of Aden system
(see inset in Figure 1).
References
-
Albarede, F. (1992). How
deep do common basaltic magmas form and differentiate? Journal
of Geophysical Research 97, 10997–11009
-
Ayele,
A., Jacques, E., Kassim, M., Kidane, T., Omar,
A., Tait, S., Nercessian, A., de Chabalier, J.
B. & King,
G. (2007). The volcano–seismic crisis in
Afar, Ethiopia, starting September 2005: Earth
and Planetary Science Letters 255,
177–187.
-
Ayalew,
D., Ebinger, C., Bourdon, E., Wolfenden, E., Yirgu,
G., & Grassineau, N. (2006). Temporal compositional
variation of early syn–rift rhyolites along the
western Red Sea margin and northern Main Ethiopian
rift. In: Yirgu, G., Ebinger, C. J. & Maguire,
P. K. H. (eds) The Afar Volcanic Province within
the East African Rift System. Geological Society
of London, Special Publication 259,
121–130.
-
Baker, J., Snee, L. & Menzies, M.
(1996). A brief Oligocene period of flood volcanism
in Yemen: implications for the duration and rate of
continental flood volcanism at the Afro–Arabian
triple junction. Earth
and Planetary Science Letters 138,
39–55.
-
Beccaluva, L., Siena, F., Coltorti,
M., Di Grande, A., Lo Giudice, A., Macciotta, G.,
Tassinari, R. & Vaccaro,
C. (1998). Nephelinitic to tholeiitic magma generation
in a transtensional tectonic setting: an integrated
model for the Iblean volcanism, Sicily. Journal
of Petrology 39, 1547–1576.
-
Beccaluva,
L., Azzouni–Sekkal, A., Benhallou,
A., Bianchini, G., Ellam, R. M., Marzola, M., Siena,
F. & Stuart, F. M. (2007a). Intracratonic asthenosphere
upwelling and lithosphere rejuvenation beneath
the Hoggar swell (Algeria): Evidence from HIMU
metasomatised lherzolite mantle xenoliths. Earth and Planetary
Science Letters 260, 482–494.
-
Beccaluva,
L., Bianchini, G. & Wilson, M. (2007b). Cenozoic
Volcanism in the Mediterranean Area. Special Paper
of Geological Society of America 418,
358 pp.
-
Beccaluva L., Bianchini G.,
Ellam R.M., Marzola M, Oun K. M., Siena F. & Stuart F.M. (2008). The
role of HIMU metasomatic components in the African
lithospheric mantle: petrological evidence from the
Gharyan peridotite xenoliths, NW Libya. In: Coltorti
M. & Gregoire,
M. (eds) Metasomatism in oceanic and continental
lithospheric mantle. Geological Society of
London, Special Publications 293,
253–277.
-
Bedini, R. M., Bodinier, J.–L,
Dautria, J.–M. & Morten,
L. (1997). Evolution of LILE–enriched small
melt fractions in the lithospheric mantle: A case
study from the East African Rift. Earth and Planetary.
Science Letters 153, 67–83.
-
Bellahsen,
N., Faccenna, C., Funiciello, F.,
Daniel, J. M. & Jolivet, L. (2003). Why did
Arabia separate from Africa? Insights from 3-D
laboratory experiments. Earth and Planetary
Science Letters 216,
365–381.
-
Bosellini, A., Russo, A., Arush,
M. A. & Cabdulqadir,
M. M. (1987). The Oligo–Miocene of Eil (NE Somalia):
a prograding coral–lepidocyclina system. Journal
of African Sciences 6,
583–593.
-
Brooker,
R. A., James, R. H. & Blundy, J. D. (2004).
Trace elements and Li isotope systematics in Zabargad
peridotites: evidence of ancient subduction processes
in the Red Sea mantle. Chemical Geology 212,
179–204.
-
Campbell, I.H., Czamanske, G.
K., Fedorenko, V.A. & Hill,
R.I. (1992). Syncronismof the Siberian traps and
the Permian-Triassic boundary. Science 258,
1760–1763.
-
Campbell, I.H. (2001). Identification
of ancient mantle plumes. In: Ernst, R.E. & Buchan,
K. L. (eds) Mantle
plumes: their identification through time. Geological
Society of America, Special Paper 352,
5–21.
-
Campbell, I.H. (2007). Testing the plume
theory. Chemical
Geology 241, 153–176.
-
Cawthorn,
R. G. & Biggar, G. M. (1993). Crystallization
of titaniferous chromite, magnesian ilmenite and
armalcolite in tholeiitic suites in the Karoo Igneous
Province. Contributions
to Mineralogy and Petrology 114,
221–235.
-
Conticelli, S., Sintoni, M.
F., Abebe, T., Mazzarini, F. & Manetti, P.
(1999). Petrology and geochemistry of ultramafic
xenoliths and host lavas from the Ethiopian Volcanic
Province: An insight into the upper mantle under
eastern Africa. Acta Vulcanologica 11,
143–159.
-
Davaille, A., Stutzmann, E.,
Silveira, G., Besse, J. & Courtillot,
V. (2005). Convective patterns under the Indo-Atlantic. Earth
and Planetary Science Letters 239,
233–252.
-
Ebinger, C. J., & Sleep
N. H. (1998). Cenozoic magmatism throughout East
Africa resulting from impact of a single plume. Nature 395,
788–791.
-
Ellam, R. M. (2006). New constraints
on the petrogenesis of the Nuanetsi picrite basalts
from Pb and Hf isotope data. Earth and Planetary
Science Letters 245,
153–161.
-
Ernst, R. E. & Buchan, K.
L. (2001). Mantle
plumes: their identification through time. Special
Paper of Geological Society of America 352,
593 pp.
-
Farmer, G. L. (2003). Continental Basaltic
Rocks. In: L. R. Rudnick (ed) Treatise on Geochemistry – Chapter
3, The Crust. Oxford: Elsevier-Pergamon,
85–121.
-
Farnetani,
C. G. & Richards, M. A. (1994). Numerical
investigations of the mantle plume initiation model
for flood basalt events. Journal of Geophysical
Resources 99, 13813–13834.
-
Farnetani,
C. G., Legras, B. & Tackley, P. J. (2002).
Mixing and deformations in mantle plumes, Earth
and Planetary Science Letters 196,
1–15.
-
Falloon, T. J., Green, D. H. & Danyushevsky,
L. V. (2007). Crystallization temperatures of tholeiite
parental liquids: Implications for the existence of
thermally driven mantle plumes. In: Foulger, G. R. & Jurdy,
D. M(eds) Plates, Plumes and Planetary Processes. Geological
Society of America Special Paper 430,
235–260.
-
Ford, C. E., Russel, D. G.,
Craven, J. A. & Fisk,
M. R. (1983). Olivine–liquid equilibria:
temperature, pressure and composition dependence
of the crystal/liquid cation partition coefficients
for Mg, Fe2+, Ca and Mn. Journal of Petrology 24,
256–265.
-
Foulger, G. R., Natland, J.
H., Presnall, D. C. & Anderson,
D. L. (2005). Plates, plumes and paradigms. Special
Paper of Geological Society of America 388,
881 pp.
-
Foulger, G. R. & Jurdy,
D. M. (2007). Plates,
plumes and planetary processes. Special Paper
of Geological Society of America 430,
pp. 997.
-
Furman, T., Bryce, J., Rooney,
T., Hanan, B., Yirgu, G. & Ayalew, D. (2006). Heads and tails:
30 My of the Afar plume. In: Yirgu, G., Ebinger, C.
J. & Maguire,
P. K. H. (eds) Structure and evolution of the East
African Rift System in the Afar Volcanic Province.
Geological Society of London Special Publication 259,
97–121.
-
Gani, N. D., Gani, M. R. & Abdelsalam,
M. G. (2007). Blue Nile incision on the Ethiopian
Plateau: Pulsed plateau growth, Pliocene uplift,
and hominin evolution. GSA
Today 17, 4–11.
-
Green, D.
H. & Falloon, T. J. (2005). Primary magmas
at mid–ocean ridges, “hotspots”,
and other intraplate setting: Constraints on mantle
potential temperature. In: Foulger, G. R., Natland,
J. H., Presnall, D. C. & Anderson, D. L. (eds) Plates,
plumes, and paradigms. Geological Society of America,
Special Paper 388, 217–247.
-
Grégoire,
M., Lorand, J. P., O’reilly,
S. Y. & Cottin, J. Y. (2000). Armalcolite–bearing,
Ti–rich metasomatic assemblages in harzburgitic
xenoliths from the Kerguelen Islands: implications
for the oceanic mantle budget of high–field
strength elements. Geochimica et Cosmochimica Acta 64,
673–694.
-
Herzberg, C. & O’Hara,
M. J. (2002). Plume associated magmas of Phanerozoic
age: Journal
of Petrology 43, 1857–1883.
-
Herzberg,
C. & Asimow, P. D. (2008). Petrology
of some ocean island basalts: PRIMELT2.XLS software
for primary magma calculation. Geochemistry Geophysics
Geosystems 9, Q09001.
-
Hofmann,
C., Courtillot, V., Feraud, G., Rochette, P., Yirgu,
G., Ketefo, E. & Pik, R. (1997). Timing
of the Ethiopian flood basalt event and implications
for plume birth and global change. Nature 389,
838–841.
-
Humayun, M., Qin, L. & Norman,
M. D. (2004). Geochemical Evidence for Excess Iron
in the Mantle Beneath Hawaii. Science 306,
91–94.
-
Kieffer, B., Arndt, N., Lapierre,
H., Bastien, F., Bosch, D., Pecher, A., Yirgu,
G., Ayalew, D., Weis, D., Jerram, D. A., Keller,
F. & Meugniot,
C. (2004). Flood and Shield Basalts from Ethiopia:
Magmas from the African Superswell. Journal of Petrology 45,
793–834.
-
Irvine, T. N. & Baragar,
W. R. A. (1971). A guide to the chemical classification
of the common volcanic rocks. Canadian Journal of Earth
Sciences 8,
523–548.
-
Le Bas, M. J., Le Maitre, R.
W. & Woolley,
A. R. (1992). The construction of the Total Alkali–Silica
chemical classification of volcanic rocks. Mineralogy
and Petrology 46, 1–22.
-
Manetti,
E., Capaldi, G., Chiesa, S., Civetta, L., Conticelli,
S., Gasparon, M., La Volpe, L. & Orsi,
G. (1991). Magmatism of the eastern Red Sea margin
in the northern part of Yemen from Oligocene to
present. Tectonophysics 198,
181–202.
-
Mège, D. & Korme,
T. (2004). Dyke swarm emplacement in the Ethiopian
Large Igneous Province: not only a matter of stress. Journal
of Volcanology and Geothermal Research 132,
283–310.
-
Melluso, L., Beccaluva, L.,
Brotzu, P., Gregnanin, A., Gupta, A. K., Morbidelli,
L. & Traversa,
G. (1995). Constraints on the Mantle Sources of
the Deccan Traps from the Petrology and Geochemistry
of the Basalts of Gujarat State (Western India). Journal
of Petrology 36,
1393–1432.
-
Merla, G., Abbate, E., Canuti,
P., Sagri, M. & Sacconi,
P. (1973). Geological Map of Ethiopia and Somalia
1:2000000. Firenze: Consiglio Nazionale delle
Ricerche (CNR).
-
Mohr, P. & Zanettin, B.
(1988). The Ethiopian flood basalt province. In:
MacDougall, J. D. (ed) Continental
Flood Basalts. Dordrecht: Kluwer, 63–110.
-
Moine,
B. N., Grégoire, M., O’reilly,
S. Y., Sheppard, S. M. F. & Cottin, J. Y. (2001).
High Field Strength Element Fractionation in the Upper
Mantle: Evidence from Amphibole–Rich Composite
Mantle Xenoliths from the Kerguelen Islands (Indian
Ocean). Journal of Petrology 42,
2145–2167.
-
Niu, Y. & Batiza, R. (1991). An
empirical method for calculating melt compositions
produced beneath mid–ocean ridges: application
for axis and off–axis
(seamounts) melting. Journal of Geophysical Research 96,
21753–21777.
-
Niu, Y. L. & O'Hara, M.
J. (2008). Global correlations of ocean ridge basalt
chemistry with axial depth: A new perspective. Journal of
Petrology 49,
633–664.
-
Piccirillo, E. M. & Melfi,
A. J. (1988). The
Mesozoic Flood Volcanism of the Parana Basin:
Petrogenetic and Geophysical Aspects. Sao
Paulo: University of Sao Paulo. 600 pp.
-
Pik, R., Deniel, C.,
Coulon, C., Yirgu, G., Hofmann, C. & Ayalew,
D. (1998). The northwestern Ethiopian Plateau flood
basalts: Classification and spatial distribution
of magma types: Journal
of Volcanology and Geothermal Research 81,
91–111.
-
Pik, R., Marty, B. & Hilton,
D. R. (2006). How many mantle plumes in Africa?
The geochemical point of view. Chemical Geology 226,
100–114.
-
Rogers, N. W., MacDonald, R.,
Fitton J. G., George, R., Smith, M. & Barreiro,
B. (2000). Two mantle plumes beneath East African
rift system: Sr, Nd, and
Pb isotope evidence from Kenya rift basalts. Earth
and Planetary Science Letters 176,
387–400.
-
Rogers, N. (2006). Basaltic
magmatism and the geodynamics of the East African
rift system. In: Yirgu, G., Ebinger, C.J. & Maguire,
P.K.H. (eds) The Afar Volcanic
Province within the East African Rift System. Geological
Societyof London, Special Publications 259,
77–93.
-
Segnör, A. M. C. (2001). Elevation
as indicator of mantle plume activity. In: Ernst,
R. & Buchan,
K. (eds.) Mantle plumes: their identification
through time. Geological Society of America
Special paper 352, 183–225.
-
Sicilia,
D., Montagner, J. P., Cara, M., Stutzmann, E.,
Debayle, E., Lépine, J. C., Levêque,
J. J., Beucler, E., Sebai, A., Roult, G., Ayele, A. & Sholan,
J. M. (2008). Shear–wave velocities and stratification
of anisotropic upper mantle structure beneath the
Afar Hotspot region. Tectonophysics 462,
164–177.
-
Sobolev, A. V., Hofmann, A.
W., Kuzmin, D. V., Yaxley, G. M., Arndt, N. T., Sun–Lin
Chung, S–L,
Danyushevsky, L. V., Elliott, T., Frey, F. A, Garcia,
M. O, Gurenko, A. A., Kamenetsky, V. S., Kerr,
A. C., Krivolutskaya, N. A., Matvienkov, V. V.,
Nikogosian, I. K., Rocholl, A., Sigurdsson, I.
A., Sushchevskaya, N. M. & Teklay, M. (2007).
The Amount of Recycled Crust in Sources of Mantle-Derived
Melts. Science 316,
412–417.
-
Sun, S. S. & McDonough,
W. F. (1989). Chemical and isotopic systematics
of oceanic basalts: implications for mantle composition
and processes. In: Saunders, A. D., Norry, M. J.
(eds.) Magmatism
in the Ocean Basins. Geological Society of
London, Special Publication 42,
313–347.
-
Sweeney, R. J., Falloon, T.
J., Green, D. H. & Tatsuni,
Y. (1991). The mantle origins of Karoo picrites. Earth
and Planetary Science Letters 107,
256–271.
-
Tolstikhin, I. N. & Hofmann,
A. W. (2005). Early crust on top of the earths
core: Physics
of the Earth and Planetary Interiors 148,
109–130.
-
Ukstins, I., Renne, P., Wolfenden,
E., Baker, J., Ayalew, D. & Menzies, M. A.
(2002). Matching conjugate volcanic rifted margins:
40Ar/39Ar chronostratigraphy of pre- and syn-rift
bimodal flood volcanism in Ethiopia and Yemen. Earth
and Planetary Science Letters 198,
289–306.
-
Yirgu, G., Ebinger, C.J., Maguire P.K.H.
(2006).
The Afar volcanic province within the East African
Rift System. Special Publication of Geological Society
of London, 327 pp.
Discussion
Jerry Winterer, 23rd November, 2009
- In this webpage, Beccaluva et al. never
get below the upper part of the asthenosphere.
The plume in the summary figure connecting to
the deeper interior is gratuitous. Everything about
the petrology works without a plume.
- The citation for doming
is Bosellini et al. (1987), which has nothing
about doming. In fact, the exposure of pre-Oligocene
strata is ascribed to a large Oligocene drop in sea
level. The Bosellini data come from the Aden coast
of Somaliland, not from the Afar region.
This article is the usual set of interesting
and valuable observations about petrology, plus completely
unrelated speculation about deep plumes tacked on,
as a non sequitor.
Back
last updated 17th
November, 2009 |