|
Bermuda
and the Bermuda Rise – A Poor Fit to
the Classical Mantle Plume Model |
Peter
R. Vogt
Marine Science Institute,
University of California at Santa Barbara, Ptr_vogt@yahoo.com
Woo-Yeol
Jung
Code 7420, Naval Research
Laboratory, Washington, DC 20375-5320
(See Vogt, P.R. & W.-Y.
Jung, Origin
of the Bermuda volcanoes and Bermuda Rise: History,
observations, models, and puzzles, in Plates,
Plumes, and Planetary Processes, G.R. Foulger
and D.M. Jurdy (Eds.), Geological Society of America
Special Paper 430, in press, for more complete coverage
of the topics discussed in this webpage).
Click here to
download a PDF version of this webpage
Introduction
The mid-plate
Bermuda volcanoes and the swell on whose crest
they reside (Figure 1), are often included
in global studies of mid-plate oceanic swells,
but they are among the most difficult for a
deep mantle plume model to explain. In fact,
Bermuda offers problems for every current model. A
comprehensive synthesis, history and review
is presented in Vogt
& Jung (2007).
The "Bermuda Islands" comprise
the small subaerial summit of a 15-100 m thick,
665 km2 cap of reef and reef-derived
carbonates, resting on the eroded stump – the
Bermuda Pedestal – of a mid-to-late Eocene
shield volcano. Three other submerged edifices,
together with Bermuda, form a NE-trending, 100-km-long
line, paralleling the plate-tectonic fabric of
the ca. 123-124 Ma oceanic crust on which
the volcanoes rose.
The volcanic line is located near
the summit of the evidently related Bermuda Rise,
a NE-trending oval swell (1500 km long and 500-1000
km wide) representing at its summit a 800-1000
m positive depth anomaly (e.g., Sclater & Wixon,
1986; Detrick et al., 1986; Sheehan
& McNutt, 1989), whose exact magnitude
depends on the oceanic crust age-vs-depth model
assumed. Associated with the depth anomaly
is a geoid high of ca. 5-10 m (Crough,
1978; Detrick et al.,
1986; Sheehan &
McNutt, 1989) over the rise summit. Taken
in concert with its depth anomaly, this geoid
high implies a compensation depth in the range
40-80 km; Sheehan & McNutt (1989)
derived 55 ± 10 km.
A
corresponding regional heat flow anomaly is at
most 5 to 10 mW/m2 (Detrick et al.,1986; Louden
et al., 1987), and recent demonstrations
of the importance of low-temperature hydrothermal
effects (e.g., Harris et al., 2000; McNutt,
2002; von Herzen, 2004; DeLaughter et
al.,
2005) makes even this value suspect.
For a mid-plate setting, the Bermuda
Rise is anomalously active sesimically, an observation Zhu
& Wiens (1991) attributed to thermoelastic
stress caused by hotspot reheating. Seismic tomography
(e.g., Zhao, 2004; Ritsema,
2005) has so far failed to detect a significant
wave-speed anomaly extending from below Bermuda
into the middle or lower mantle.
|
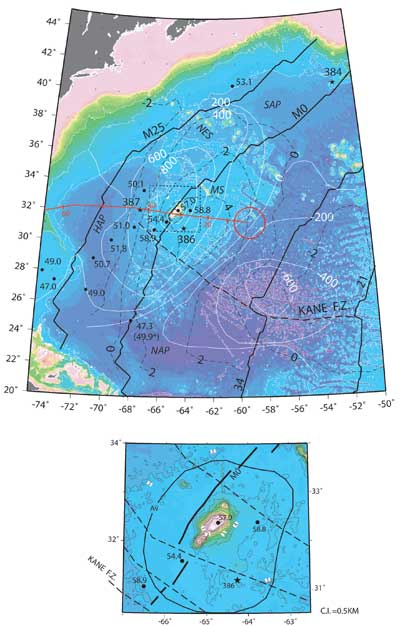
Figure
1. Top: Greater Bermuda Rise region: Bathymetry
(Smith
& Sandwell, 1997), key magnetic lineations
(Mueller et al., 1993), DSDP Leg 43 drill
site locations (stars); heat flow values (Detrick
et al., 1986; Louden et al., 1987);
residual depth anomaly (white contours at 200
m interval; Sclater & Wixon,
1986); residual geoid anomaly (meters; dashed
black lines); trace of Kane Fracture Zone (dashed
line, after Jaroslaw
& Tucholke, 1994); and predicted track (red)
of North America plate over a fixed Bermuda hotspot
(Duncan et al., 1984), with predicted present
hotspot location shown by large red circle. Heat
flow station on southern BR shows the value 47.3
mW/m2 calculated by Detrick et al.
(1986), recalculated to 49.9 mW/m2 by
Louden et al. (1987) using the same data.
NES, New England Seamounts; SAP, Sohm Abyssal
Plain; HAP, Hatteras Abyssal Plain; NAP, Nares
Abyssal Plain; MS, Muir Seamount. Bottom: Bathymetry
(Smith & Sandwell,
1997) of Bermuda and vicinity, with heat flow
stations (W/m2) from Detrick et
al. (1986)
and Hyndman et al. (1974), location of DSDP
site 386 (The Shipboard Party, 1979), and sea-floor
spreading magnetic anomaly M-0 from Klitgord
& Schouten (1986). Solid line shows
outer limit of seismic reflector Av – caused
by volcanogenic sediments of Bermudan origin
(Tucholke
& Mountain, 1979). Dashed lines indicate
major fracture zones as interpreted from geophysical
data. |
Age dating
Age-dating the Bermuda
volcanism and rise development plays a crucial role
in testing any model, especially the stationary deep
mantle plume model. Boreholes on Bermuda, as well as
DSDP Site 386 located in a fracture valley 140 km SE
of Bermuda (Tucholke & Vogt, 1979) indicate
that submarine eruption building up the shield began
no later than the late Middle or early Late Eocene,
when erosional debris from the emergent edifice first
arrived at Site 386. While K-Ar whole-rock dates of 52
Ma (1958 borehole; Gees, 1969) and 47
and 91 ± 5 Ma (1972 borehole; Reynolds
& Aumento,
1974) were reported for these pillow lavas, their
alteration makes these dates very unreliable. In
a later igneous episode, highly titaniferous dikes
(sheets) were intruded into the edifice. However,
the sheets have yielded consistent and credible dates of 33-34
Ma, confirmed by recent Ar-Ar dating reported by
Williamson et al. (2006). Phlogopite weathered
out of the eroding sheets first arrived at Site 386
(The Shipboard Scientific Party, 1979) in
the middle to upper Oligocene (ca 30-25 Ma), consistent
with the radiometric dates. The initiation of Bermuda
Rise uplift has been stratigraphically dated (early
to middle part of the Middle Eocene, i.e., ca 48-45
Ma; The Shipboard Scientific
Party, 1979; Tucholke
& Vogt, 1979) by the cessation of biogenic
turbidites that had covered the central and western
parts of the present rise area. The initiation of
igneous activity and uplift may therefore have been
coeval. However, while evidence to date suggests
Eocene shield formation was succeeded by 33-34 Ma
intrusions, analysis of seismic reflection returns
from successively tilted turbidites in the Kane Fracture
Zone valley suggests that Bermuda Rise uplift continued
into Miocene, with 400-500 m of uplift occurring
after the sheet intrusion phase.
A Bermuda Plume?
Bermuda ranks very
low when observational data are compared to predictions
of the mantle plume model (Courtillot
et al., 2003;
Anderson,
2005). Both the 100-km-long volcanic
lineament and the 1500-km-long Bermuda Rise trend northeast,
nearly at right angles to the trace predicted from
North America "absolute" motion
models (Figures 1 and 2; Morgan, 1983; Duncan,
1984;
Mueller et al., 1993). Bathymetry shows no
evidence for any geologically young igneous activity
along the predicted trace, which extends east 650
km from Eocene Bermuda to the predicted present site
of a putative Bermuda hotspot (Vogt
& Jung,
2007).
Moreover, Jaroslaw & Tucholke (1994) found
no evidence for migratory uplift associated with the
Bermuda Rise. The shallow depth of volcanic basement
under Bermuda shows that little or no subsidence has
occurred. Morgan & Crough (1979), who were aware of
the lack of a hotspot trace, proposed a causal relation
between uplift of the Cape Fear Arch on the North American
margin, and the passage of the plate over a Bermuda
plume; however, more recent studies of this arch are
inconsistent with this idea. Those authors also related
Cretaceous kimberlites and other igneous rocks in Eastern
North America to the passage of the plate over a Bermuda
plume.
Some authors (e.g.,
Cox & Van Arsdale, 2002) have held on
to the Morgan-Crough Bermuda plume model, which would
require a a pulsating (“lava
lamp”) plume and/or severe control by lithospheric
structure on melts rising into or erupting onto the
crust. In support, Cox &
Van Arsdale (2002)
note that predicted Bermuda hotstpot tracks cross the
ca. 65 Ma igneous activity in Mississippi, and also
the ca 115 Ma activity in Kansas (Figure 2). However,
there is no “LIP” (large igneous plateau)
in Kansas or elsewhere that might represent the effects
of a “plume head” at the beginning
of the putative Bermuda plume track. Moreover,
Cretaceous and Cenozoic igneous rocks elsewhere in
North America (some are noted in simplified form in
Figure 2) would necessarily require other plumes
(McHone,
1996) [Ed: See also CAMP page].
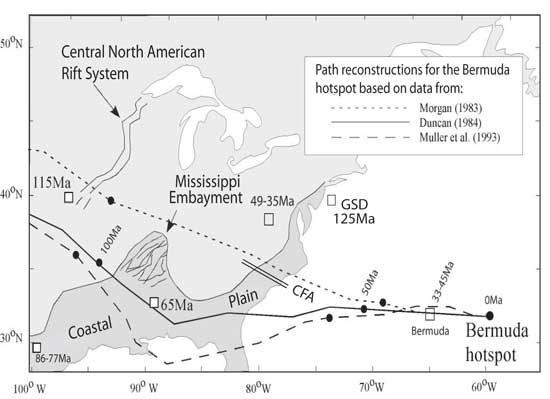
Figure 2. Alternative
traces of the Bermuda “hotspot” relative
to the North America plate, as predicted by the plate/mantle
motion models of Morgan (1983), Duncan (1984) and Mueller
et al. (1993), with filled circles indicating the predicted
hotspot location at 0, 50 and 100 Ma for each trace.
Thin lines within the Mississippi Embayment are the
Mississippi Valley graben faults, and open squares
show the average location of the earliest (ca. 115
Ma) and latest (ca. 65 Ma) eruptions along a migratory
path of igneous activity. CFA and GSD denote Cape Fear
Arch and Great Stone Dome. Modified from Figure 1 of
Cox & van Arsdale (2002).
Models
A number of attempts
have been made – mostly in the late 1970s-1980s –
to model the formation and present state of the Bermuda
Rise, in many cases along with some other, similar
mid-plate swells (e.g., Crough, 1978; Sclater
& Wixon, 1986; Detrick
et al., 1986; Louden et al.,
1987; Liu & Chase, 1989; Sheehan
& McNutt, 1989).
In any case, the geoid and depth anomalies require
the Bermuda Rise to be supported by a low density root
at depth within the lithosphere or/and upper asthenosphere
– it is not thickened oceanic crust. If anything, the
crust under the Bermuda Rise (except at the Bermuda
volcanoes) is anomalously thin (Lizzaralde
et al.,
2004). Little or no low-seismic-wave-speed anomaly
has been detected by tomography in the mantle
below Bermuda (Zhao, 2004). The lack of current
subsidence and the low or nonexistent heat flow anomaly
have been problematic for most models. However, published
models suggest that certain combinations of thermal
expansion, dynamic uplift, and perhaps residual lower-density
melt accumulation below the rise, may account for observations.
(The latter was proposed by Phipps
Morgan et al., 1995,
for the Hawaii and Cape Verde "hotspots").
Thermal expansion by itself cannot explain the rise
except in combination with convection – purely
conductive models require too high a temperature anomaly. Some
of the models have to be rejected or at least refined
to account for continuous but non-migratory Eocene-Miocene
uplift (Jaroslaw & Tucholke, 1994) and possible lack of a mantle-related
heatflow anomaly.
Non-plume
alternatives
If
Bermuda was not formed by a pulsating lava-lamp-type
plume, what can account for the observations? Whatever
is happening under Bermuda is evidently traveling with
the North America plate. Sclater
& Wixon (1986), Vogt (1991), King
& Anderson (1998) and King (2007)
[Ed: See also EDGE page] have examined an "edge-driven" convection
that models predict should be generated where thick,
cold continental lithosphere abuts an oceanic plate,
once the ocean has widened sufficiently. The models
predict rising convection currents ca. 1000 km seawards
of the boundary. The persistent Eocene to Miocene uplift
of the Bermuda Rise, as well as the rise location and
orientation, are qualitatively consistent with edge-driven
convection.
Vogt
& Jung (2007) note
that Bermuda Rise formation and volcano formation
were coeval, within error bounds, with the Hawaii-Emperor
Bend (long dated at ca 43 Ma, but recently redated
to ca. 50 Ma; Sharp
& Clague,
2006). This and other coeval events might represent
an abrupt rearrangement of mantle convection, plate
motions, and intra-plate stresses triggered
by the closing of the Tethys at about that time. Such
a global rearrangement has been suggested by Rona
& Richardson (1978), among others. Alternatively
or relatedly, slabs accumulating near the 660-km discontinuity
may have sunk into the lower mantle at that time (Fukao
et al., 2001), triggering rearrangements of mantle
flow.
While
such a global change might have triggered the "Bermuda
event", what can explain its geographic location
and rise orientation? Edge-driven convection (e.g.,
King, 2007) can explain this, as noted. "Lithosphere
pre-conditioning" is another (Vogt
& Jung,
2007).
Both the rise and the volcanic lineament parallel the
structure of the underlying oceanic crust, i.e. the
isochrons. The rise was developed in crust and mantle
lithosphere known to have been formed at very low spreading
rates, and exhibiting the rough basement typical of
slow spreading. Lizzaralde et
al. (2004) found this
crust to be anomalously thin, with the underlying mantle
atypical. This led Vogt & Jung (2007) to
speculate that the nature of the mantle lithosphere
may have made it more vulnerable to partial melting
at depth. Alternatively, the mantle below the North
American plate that happened to underlie the Bermuda
rise region during the Eocene
might have been anomalous in composition and/or temperature.
Future
research opportunities
How can
future research discriminate among the wealth of
models so far presented in the literature, and
perhaps others still to be developed? First, earthquake-source
seismic tomography with even better resolution
than e.g., that presented in Fig. 19 of Zhao (2004)
may delineate a "slow" region
in the upper 400 km of the mantle below the Bermuda
Rise. Below some such depth, the mantle must be decoupled
from the motion of the North America plate. Explosion-source
seismic experiments with OBS arrays on the rise, expensive
and perhaps not feasible due to concerns for marine
mammals, would probably be needed to test for a buoyant
refractory root (e.g., Phipps
Morgan et al., 1995;
Holm, 2006) which might extend from ca. 50
km (the depth of swell compensation deduced from geoid
data) to 200 km below the rise. (The depth of origin
of the Bermuda sheets is ≥ 150 km; Olsen,
2005). Airgun-source multichannel profiling of the
type conducted by Lizarralde et al. (2004)
across the southern Bermuda Rise might detect cooled
intrusions which reached the upper mantle lithosphere.
However, any reduced wave-speed anomalies would have
to allow for melt retention under the slow spreading
rates forming most of the crust under the Bermuda Rise
(Lizarralde et al., 2004). Spreading-rate-dependent
mantle velocities should change only gradually northwards
along isochrons, whereas any anomalies associated with
the Bermuda Rise should reach extrema under the rise
summit. Future deep seismic experiments in the ocean
surrounding Bermuda are influenced by concerns – whether
scientifically justified or not – that the airgun
sources will adversely impact marine mammals, particularly
whales.
Additional drilling into
the igneous basement of Bermuda and its three smaller
satellites (Plantagenet/Argos and Challenger banks,
and Bowditch seamount) is essential. Given the geologic
complexity we know from volcanic islands (e.g., the
Cape Verde archipelago; Holm et
al., 2006), it seems
highly unlikely that even the Bermuda edifice was formed
by a simple two-stage process of an Eocene tholeiitic
shield, followed after ca. 6-12 Ma of quiescence by
the 33-34 Ma "Bermudite" (Aumento & Gunn,
2006) sheet intrusions.
Further, we have assumed
– with no direct evidence! – that the three
satellite edifices are of the same age as Bermuda. This
remains pure speculation until they (and/or their flanking
debris aprons) are cored,
preferably to several km depth. A detailed magnetic
and gravity survey of all four edifice summits and
upper flanks should be conducted in advance of any
drilling or further deep-tow, AUV, or manned submersible
investigation, to help map the structural layout
of intrusive sheets, lava accumulations, central conduits
and flanking volcaniclastic debris aprons. We have
proposed such work in cooperation with the Bedford
Institute of Oceanography (Drs. Steve Blasco and Marie-Claude
Williamson).
Several deep holes similar
to those drilled at DSDP Site 386 (Figure 1) and 385
(New England Seamounts; Tucholke
& Vogt, 1979) should
also be placed around the bases of the four Bermuda
edifices, as close as possible to the bases, but still practically penetrating
the volcaniclastic debris and flows (i.e. the "inner" seismic reflector
Av of Tucholke & Mountain, 1979), to recover and biostratigraphically
date the youngest sediments overlain by the oldest Bermudan rocks. Recovery of
larger, less altered rock fragments would also be more likely closer to the base
of the volcanic edifices.
A transect of a few boreholes
across and along the Bermuda Rise, just deep enough
to sample the oldest hemipelagic sediment just above
the Eocene biosiliceous turbidites, should be able
to resolve
the detailed spatial-temporal pattern of BR uplift
initiation. Such boreholes might also recover the time
when bottom currents were first steered by the BR (e.g., Ayer
& Laine,
1982). Several boreholes should be placed along the
Kane fracture zone to calibrate the uplift history
deduced by Jaroslaw & Tucholke (1994)
from seismic reflection mapping of more local turbidites.
Abyssal plains, with gradients of 1:1000 or less (the
present BR
is surrounded on three sides by modern or at least
late Pleistocene abyssal plains; Pilkey
& Cleary, 1986), should be extremely sensitive
to small elevation changes. The Mid-Eocene turbidite
offlap pattern – in time and space – would
depend on the uplift mechanism.
A plume model predicts
rise uplift migrating radially outwards from a region
above the upwelling plume head, whereas a "distributed
source" model
with simultaneous partial melting or/and temperature
increase would predict simultaneous uplift over the
entire area of the present Bermuda Rise. A plume-type
model (Griffiths & Campbell, 1991; Campbell,
2006) predicts a possible lag of about 2 Ma between
the first uplift above the center of the plume head,
and uplift on the outer fringes of the expanding asthenosphere
(I. Campbell, personal communication, 2005). Such
a lag should be recorded by the
offlapping turbidites, and is probably measurable from biostratigraphic dating
of the first hemipelagics deposited on the last turbidites. The Campbell (2006) model
also predicts a lag of ca 2-4 Ma between uplift initiation
and onset of volcanism. Current dating (Middle Eocene for onset of uplift; late
Middle to early Late Eocene or earlier for the volcanism) make such a lag possible,
but not proven. The plume model also predicts a possibly testable early central
uplift several hundred meters higher in the beginning, before spreading below
the plate flattens the head and reduces swell height during the next ca. 2 Ma. The "swell
root spreading" models of Phipps
Morgan et al. (1995;
their Fig.6 ) make even more specific and testable
predictions about rise uplift and swell radius as a
function of time.
Uplift resolution might
be further refined by correlation of individual turbidites
from one borehole to the next. Some of the thicker
and compositionally distinctive Quaternary turbidites
have been correlated from one core to another in modern
abyssal plains surrounding Bermuda (Pilkey
& Cleary,
1986). Readers interested in joining the authors in
proposing the Bermuda Rise borehole transects discussed
above are welcome. Please email us about your interest!
Acknowledgments
We
thank Marie-Claude Williamson for sharing results
from her reanalysis of Bermuda Deep Drill-72 igneous
rock samples from Bermuda. More complete acknowledgments
are given in Vogt & Jung (2007).
References
-
Anderson, D.L., Scoring hotspots: The plume and plate
paradigms, in Foulger, G.R., Natland, J.H., Presnall,
D.C., and Anderson, D.L., eds., Plates,
plumes, and paradigms, Geol. Soc. Amer. Special Paper 388, 31-54,
2005.
-
-
Aumento, F., P.H. Reynolds, and B.M. Gunn, The
Bermuda Seamount; a reactivated section of an older
oceanic crust (Abs.), Eos Trans.
Amer. Geophys. Union,
55, 455, 1974.
-
Ayer, E.A., and E.P. Laine, Seismic stratigraphy
of the northern Bermuda Rise, Mar.
Geol., 49, 169-186,
1982.
-
-
-
Cox, R.T., and R.B. Van Arsdale, The Mississippi
Embayment, North America; a first order continental
structure generated by the Cretaceous superplume
mantle event, J. Geodynamics, 34, 163-176, 2002.
-
Crough, S.T., Thermal origin of mid-plate hotspot
swells, Geophys. J. R. Astron.
Soc., 55, 451-470,
1978.
-
DeLaughter, J.E., C.A. Stein, and S. Stein, Hotspots:
A view from the swells, in Foulger, G.R., Natland,
J.H., Presnall, D.C., and Anderson, D.L., eds., Plates,
Plumes and Paradigms, Geol. Soc. Amer. Spec.
Paper 388, 257-278, 2005, doi:10.1130/2005.2388 (16).
-
Detrick, R.S., P.R. Von Herzen, B. Parsons, D.
Sandwell, and M. Dougherty, Heat flow observations
on the Bermuda Rise, J. Geophys.
Res., 91, 3701-3723,
1986.
-
Duncan, R.A., Age progressive volcanism in the
New England Seamounts and the opening of the central
Atlantic Ocean, J. Geophys.
Res., 89, 9980-9990,
1984.
-
Fukao, Y., Widiyantoro, S., and Obayashi, M., Stagnant
slabs in the upper and lower mantle transition region,
Rev. Geophys., 39, 291-323, 2001.
-
Gees, R.A., The age of Bermuda Seamount, Maritime
Sediments, 5, 56-57, 1969.
-
Griffiths, R.W., and I. Campbell,
Interaction of mantle plume heads with the Earth’s
surface and onset of small-scale convection, J.
Geophys. Res.,
96, 18295-18310, 1991.
-
Gurriet, P., A thermal model
for the origin of post-erosional alkalic lava, Hawaii,
Earth Planet. Sci. Lett., 82, 153-158, 1987.
-
Harris, R.N., R.P. Von Herzen, M.K. McNutt, G.
Garven, and K. Jordahl, Submarine hydrology of the
Hawaiian archipelagic apron, Part 1: Heat flow patterns
north of Oahu and Maro Reef, J.
Geophys. Res., 105,
21353-21370, 2000.
-
Holm, P.M., J.R. Wilson, B.P. Christensen, L. Hansen,
S.L. Hansen, K.M. Hein, A.K. Mortensen, R. Pedersen,
S. Plesner, and M.K. Runge, Sampling the Cape Verde
mantle plume: Evolution of melt compositions on Santa
Antao, Cape Verde Islands, J.
Pet., 47, 145-189,
2006.
-
Hyndman, R.D., G.K. Muecke, and F. Aumento, Deep-Drill-1972,
Heat flow and heat production in Bermuda, Can.
J. Earth Sci., 11, 809-818, 1974.
-
Jaroslow, G.E., and B.E. Tucholke, Mesozoic-Cenozoic
sedimentation in the Kane Fracture Zone, western
North Atlantic, and uplift history of the Bermuda
Rise, Geol. Soc. Amer. Bull., 106, 319-337, 1994.
-
-
Klitgord, K.D., and H.
Schouten, Plate kinematics of the central Atlantic,
in Vogt, P.R., and B.E. Tucholke, eds., The Geology
of North America, v.
M, The Western Atlantic Region, Geological Society
of America, Boulder, 351-378, 1986.
-
Liu, M., and C.G. Chase, Evolution of midplate
hotspot swells; numerical solutions, J.
Geophys. Res., 94, 5571-5584, 1989.
-
Lizarralde, D., J.B. Gaherty, J.A. Collins, G.
Hirth, and S. Kim, Spreading-rate dependence of melt
extraction at mid-ocean ridges from mantle seismic
refraction data, Nature, 432, 744-747, 2004.
-
Louden, K.E., D.O. Wallace, and R.C. Courtney,
Heat flow vs age for the Mesozoic Northwest Atlantic
Ocean: Results from the Sohm abyssal plain and implications
for the Bermuda Rise, Earth
Planet. Sci. Lett., 83,
109-122, 1987.
-
McHone, J.G., Constraints on the mantle plume model
for Mesozoic alkaline intrusions in northeastern
North America, Can. Mineralogist, 34, 325-334, 1996.
-
McNutt, M.K., Heat-flow variations over the Hawaiian
Swell controlled by near-surface processes, not plume
properties, in Hawaiian Volcanoes:
Deep Underwater Perspectives, Geophys. Monograph 128, American Geophysical
Union, Washington, DC, 355-364, 2002.
-
Morgan, W.J., and S.T. Crough, Bermuda hotspot
and the Cape Fear Arch, Eos
Trans. Amer. Geophy. Union, 60, 392-393, 1979.
-
Morgan, W.J., Hotspot tracks and the early rifting
of the Atlantic, Tectonophysics, 94, 123-139,1983.
-
Mueller, R.D., J-Y. Royer, and L.A. Lawver, Revised
plate motions relative to hotspots from combined
Atlantic and Indian Ocean hotspot tracks, Geology,
21, 275-278, 1993.
-
Olsen, S.D., Petrogenesis of Bermuda's igneous
basement (M.S. Thesis), Univ of Copenhagen, 254 pp.,
2005.
-
Phipps Morgan, J., W.J. Morgan,
and E. Price, Hotspot melting generates both hotspot
volcanism and a hotspot swell? J. Geophys. Res., 100, 8045-8062, 1995.
-
Pilkey, O.H., and W.J. Cleary, Turbidite sedimentation
in the northwestern Atlantic Ocean basin, in Vogt,
P.R. and B.E. Tucholke, eds., Turbidite
sedimentation in the northwestern Atlantic Ocean
basin, v. M, The
Western North Atlantic Region, Geology of
North America, Geol. Soc. Amer., Boulder, 437-450,
1986.
-
Reynolds, P.R., and F.A. Aumento, Deep Drill 1972:
Potassium-argon dating of the Bermuda drill core,
Can. J. Earth Sci., 11, 1269-1273, 1974.
-
Ritsema, J., Global seismic structure maps, in
Foulger, G.R., Natland, J.H., Presnall, D.C., and
Anderson, D.L., eds., Plates,
plumes, and paradigms,
Geol. Soc. Amer. Special Paper 388, 11-18, 2005.
-
-
Rowe, M.P., 1998, An explanation of the Geology
of Bermuda, Bermuda Government, Ministry of the Environment,
30 pp.
-
Sclater, J.G., and L. Wixon, The relationship between
depth and age and heat flow and age in the western
North Atlantic, in Vogt, P.R., and B.E. Tucholke,
eds. The western North Atlantic
region, v. M, The
Geology of North America, Geol. Soc. Amer., Boulder,
Colorado, 257-270, 1986.
-
-
Sheehan, A.F., and M.K. McNutt, Constraints on
thermal and mechanical structure of the oceanic lithosphere
at the Bermuda Rise from geoid height and depth anomalies,
Earth Planet. Sci. Lett., 93, 377-391, 1989.
-
The Shipboard Scientific Party, Site 386: Fracture
valley sedimentation on the central Bermuda Rise,
in Tucholke, B.E., and P.R. Vogt, eds., Initial
Reports of the Deep Sea Drilling Project, v. 43, U.S. Gov't.
Printing Office, Washington, D.C., 195-321, 1979.
-
Smith, H.F., and D.T. Sandwell, Global seafloor
topography from satellite altimetry and ship depth
soundings, Science, 277, 1956-1962, 1997.
-
Tucholke, B.E., and G.S. Mountain, Seismic stratigraphy,
lithostratigraphy, and paleosedimentation patterns
in the North American Basin, in Talwani, M, W. Hay,
and W.B.F. Ryan, eds., Deep
Drilling Results in the Atlantic Ocean: Continental
Margins and Paleoenvironment,
Maurice Ewing Ser., v. 3, Amer. Geophys.
Union, Washington, DC, 58-86, 1979.
-
Tucholke, B.E., and P.R. Vogt, Western North Atlantic:
Sedimentary evolution and aspects of tectonic history,
in Tucholke, B.E. and P.R. Vogt, eds., Initial
Reports of the Deep Sea Drilling Project, v. 43, U.S. Gov't.
Printing Office, Washington, D.C., 791-825, 1979.
-
Vacher, H.L., Rowe, M.P., and Garrett, P., 1989,
The Geological Map of Bermuda, Scale 1:25,000, Oxford
Cartographers, London, Bermuda Government, Ministry
of Works and Engineering.
-
Vogt, P.R., Bermuda and Appalachian-Labrador rises;
common non-hotspot processes?, Geology, 19, 41-44,
1991.
-
Vogt, P.R., and G.L. Johnson, Cretaceous sea-floor
spreading in the western North Atlantic, Nature,
234, 22-25, 1971.
-
Vogt, P.R., and W.-Y. Jung, Origin of the Bermuda
volcanoes and Bermuda Rise: History, Observations,
Models, and Puzzles, in Foulger, G., and Jurdy, D.,
eds., Plates, Plumes, and Planetary
Processes, Geological
Society of America Special Paper 430, 2007 (in press).
-
Von Herzen, R.P., Geothermal
evidence for continuing hydrothermal circulation
in older (>60 my) ocean crust, in Davis, E.E.,
and E.H. Elderfield, eds., Hydrogeology
of the oceanic lithosphere, Cambridge Univ. Press, Cambridge,
414-447, 2004.
-
Williamson, M.-C., M. Villeneuve, and S. Blasco,
Deep Drill 1972 revisited: New data on the composition
and age of the Bermuda volcanic edifice (Abs.), Eos
Trans. Amer. Geophys. Union, 2006.
-
Zhao, D., Global tomographic images of mantle plumes
and subducting slabs: insight into deep Earth dynamics,
Phys. Earth and Planet. Int., 146, 3-34, 2004.
-
Zhu, A., and D.A. Wiens, Thermoelastic stress in
oceanic lithosphere due to hotspot reheating, J.
Geophys. Res., 96, 18323-18334, 1991.
last updated 2nd
August, 2007 |