 |
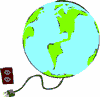 |
Energetics
of the Earth and the Missing Heat Source Mystery
|
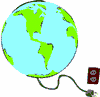 |
|
|
Overview
Global
heat flow estimates range from 30 to 44 TW (Table
1a; Reference List A). Estimates of the radiogenic
contribution (from the decay of U, Th and K in the
mantle), based on cosmochemical considerations,
vary from 19 to 31 TW (Table 1b). Thus, there is
either a good balance between current input and
output, as was once believed (“the Chondritic
Coincidence”), or there is a serious missing
heat source problem, up to a deficit of 25 TW. Attempts
to solve the perceived deficit problem include invoking
secular cooling and deep, hidden heat-source layers
(e.g., Kellogg et al., 1999).
In
many studies it has been assumed that there should
be a steady-state balance, or close to it, between
current radioactive heat production in the mantle
and current heat flow, and that very little heat
is generated in the upper mantle. This view ignores
mass balance considerations, other sources of energy
(Reference List C), secular cooling, delays in the
system, and the wide range of radioactive contents
of upper mantle materials. Among the problems commonly
cited with various models of mantle thermal history
are very high mantle temperatures in the Archaean,
survival of ancient cratonic roots, komatiitic temperatures,
over-heating of the lower mantle, freezing of the
core, an imbalance between the helium and heat flow
budgets and a perceived missing heat source. A common
perception is that there is not enough radioactivity
in the upper mantle to provide a significant contribution
to current heat flow. These problems can be avoided
by recognizing that:
- heat flow is a three-dimensional
problem and that heat flow is diverted into the ocean
basins;
- continents tend to move towards
cold downwelling mantle;
- at high mantle temperatures water
is removed from both the mantle and the lithosphere,
stiffening the system;
- the mantle is probably chemically
layered, extending the cooling time, and
- the upper mantle cannot be entirely
composed of ultradepleted
MORB and barren peridotite.
It
is useful to compile the sources of energy and global
heat flow before addressing perceived problems.
It is also useful to investigate possible shortcomings
of various theoretical models. It turns out that
the unknown hydrothermal contribution to cooling
of old oceanic lithosphere and the temperature dependence
of thermal conductivity are key issues (Hofmeister
& Criss,
2003). If lattice and radiative conductivity
are as high as currently calculated, heat that leaves
the core is mostly conducted down the core adiabat
(Gubbins, 1977), and contributes to the bulk
mantle energy budget, rather than be piped directly
to the surface e.g., in plumes (Stacey &
Stacey, 1999). The present-day heat flow through
the surface of the Earth is consistent with energy
sources in the interior, including secular cooling,
the gravitational contraction associated with cooling,
and decline of radioactive abundances. Theoretical
corrections to observed heat flow, and theoretical
estimates of expected oceanic heat flow are both
uncertain and model-dependent. Within the uncertainties
of data and theory, there is no missing heat source
paradox or need for substantial contribution to
observed heat flow from the deep mantle.
|
Distribution of radioactive elements
Radioactive
decay is only one of the sources of mantle heat
flow (Reference List C). In most current models
of geodynamics and geochemistry the lower mantle
is assumed to have escaped accretional differentiation
and to retain primordial values of radioactivity
and noble gases. The crust is assumed to have been
derived from only the upper mantle, making it extraordinarily
depleted in radioactive and volatile elements. The
heat productivity of the most U-poor mid-ocean ridge
basalts are taken as upper bounds on the heat productivity
of the whole mantle above the 650-km phase change.
This combination of assumptions, plus neglect of
non-radiogenic sources of heat, have led to the
view by some that there is a missing energy source
in the Earth.
Mass
balance calculations and the 40Ar content
of the atmosphere show that most, if not all, of
the mantle must have been processed and degassed
in order to explain the concentrations of incompatible
and volatile elements in the outer layers of the
Earth (Reference List B). The planetary accretional
zone-refining process results in an outer shell
that contains most of the U, Th and K, at a level
about three times chondritic (if the outer shell
is equated with the present mantle above 650 km),
from which the proto-crust and basaltic reservoirs
were formed. The residual (current) upper mantle
retains radioactive abundances greater than chondritic
while the bulk of the mantle, including the lower
mantle, is essentially barren (Anderson,
1989). The outer shells of Earth contain both depleted
and enriched sources and probably also contain the
bulk of the terrestrial inventory of noble gases
(Meibom & Anderson, 2003).
In
a layered model the delay between heat generation
and conduction through the surface, and the secular
cooling of the Earth, extends the thermal evolution.
As the mantle cools, it becomes less molten, degasses
less readily and probably becomes more volatile-rich,
since it is now a sink for CO2 and water.
It thus experiences different styles of convection
and cooling. These factors are not considered in
currently popular models used by convective modelers,
leading to apparent paradoxes.
In
other recent models the entire mantle is assumed
to have escaped chemical differentiation, except
for crust extraction, and to convect as a unit,
with material circulating freely from top to bottom.
Recycled material is quickly stirred back into the
whole mantle. In these models:
-
chemical
heterogeneities are embedded in a depleted matrix,
-
the whole
mantle is treated as uniformly heterogeneous,
and
-
the mantle
is relatively cold and the hot thermal boundary
layer above the core plays an essential role in
piping heat to the surface.
Convection is
assumed to be an effective homogenizer. Recent amendments
to this idea assert that there must be a radioactive-rich
layer deep in the mantle, but this is based on unlikely
assumptions about upper mantle radioactivity. Most
convection calculations ignore accretional differentiation
and the effects of pressure and temperature on thermal
properties such as thermal expansion and thermal conductivity.
Current models assume that heat from the core and
heat from the mantle are decoupled in the sense that
plumes remove core heat and plate tectonics removes
mantle heat (e.g., Stacey, 1992).
Although
there is no missing energy when direct heat flow
data are used, there is a mismatch between oceanic
heat flow and theoretical predictions from the cooling
plate model. In some compilations, about 12 TW is
added to the measured global heat flow to match
the square-root-of-age predictions. This is the
same size as the perceived “missing”
heat energy (Hofmeister
& Criss,
2003).
Tables
1a & b summarize estimates of global heat flow
and heat sources in the mantle (Reference Lists
A & C). The references are subdivided by subject.
See also other sections on heat flow and temperature
at http://www.mantleplumes.org
|
Heat
losses
Components
and mechanisms of heat loss
Heat flows
through the interior of the Earth by radiation, conduction
and convection. Eventually, all heat flows through the
surface boundary layer, primarily by lattice conductivity
but some by dikes, volcanoes and hydrothermal activity.
The surface
boundary condition has changed with time. As a planet
cools it evolves from a magma ocean regime (the result
of accretional heating and gravitational differentiation),
to a thick buoyant surface layer (unsubductable basalt
and refractory residue) with heat pipes and heat sheets
(permeable plates), to plate tectonics, and finally
to stagnant lid. The present continental crust, asthenosphere
and olivine-rich (pyrolitic) upper mantle are most likely
reprocessed residues from early differentiation. Currently,
the surface boundary layer is a conduction boundary
layer with an average thickness of 100-200 km. It is
pierced in places by volcanoes that deliver a relatively
small amount of heat to the surface via magma. The cooling
of the mantle is mainly accomplished by the cooling
of the surface plates.
In early Earth
history a transient magma ocean allowed magmas to transfer
their heat directly to the atmosphere. As buoyant material
collected at the top, the partially molten interior
became isolated from the surface. Magma, however, could
break through and create “heat pipes” to
carry magma and heat to the surface. Io and Venus may
utilize this mechanism of heat transfer. The surface
boundary condition in these cases can be viewed as a
permeable plate. Present day plates can be penetrated
by sills and dikes and are therefore partially permeable.
As a planet cools further it may jump to a stagnant-lid
state with a convecting interior. Mars and Moon may
be in such a state.
Currently,
the Earth’s interior is cooling by a combination
of thermal conduction through the surface and the
advection of cold material to the interior by slabs
and delaminated continental crust. The heat generated
in the interior of the Earth, integrated over some
delay time, is transferred to the surface conduction
boundary layer by a combination of solid-state convection,
fluid flow, radiation and conduction. Crustal radioactivity
is a major contributor to continental heat flow.
Delaminated crustal blobs may contain much of the
radioactivity in the mantle.
In the ocean
basins the main contribution to the observed heat flow
is the transient effect of the formation of the oceanic
crust (Reference List F). Theoretically, this conducted
heat flow should wane as the square-root of age but
surprisingly, it is nearly constant. The background
heat flow is nearly the same as under continents. In
contrast to predictions from the plate and cooling half-space
models there is little correlation of heat flow with
age or depth of the ocean. There is also little evidence
that hotspots or swells are associated with high heat
flow (see Heatflow page;
Stein
& Stein, 2003). This indicates that the underlying
mantle is not isothermal or homogeneous.
Thermal conductivity
decreases rapidly with increasing temperature. The cold
outer shell of the Earth is not simply a cooling boundary
layer of uniform composition and conductivity, losing
heat by conduction alone, as assumed in cooling-plate
and half-space models (Reference List F). The fact that
heat flow is not a function of square-root of age suggests
that some process affects the near-surface thermal gradient
without affecting the integrated density of the outer
layers. Oceanic swells, on the
other hand, apparently require a redistribution of
mass and density. They do not appear to be purely thermal
in origin. They are probably largely held up by the
buoyancy of depleted harzburgite (the perisphere).
Heat
loss from the continents
The mean heat
flow from continents is about 80 mW/m2 (Reference
List A). The heat flow that can be attributed to the
continental crust itself is about half of this, or 32-40
mW/m2, though recent estimates of the average
U, Th and K concentrations vary by almost a factor of
2. The other half thus comes from the mantle. The continental
crust therefore accounts for 5.8 - 8 TW of the global
heat flow.
Heat
loss from the oceans
Because of sparse
coverage, heat flow data must be averaged by age and
by area of the seafloor. These estimates give about
62 mW/m2 for the average oceanic heat flow
(Hofmeister
& Criss,
2003). About half of this is a transient effect
from the plate-forming process and half is the background
flux from the mantle. Measured oceanic heat flow varies
from about 300 to 25 mW/m2 with 45 to 55
mW/m2 being a representative range through
old oceanic crust. The theoretical value for half-space
cooling is ~ 101 mW/m2 (Pollack et al.,
1993) but this is sensitive to values adopted for thermal
conductivity of the mantle and crust. Theoretical plate
cooling model values are infinity at zero age and 100
mW/m2 at 30 Ma. The theoretical value at
large time depends on preselected parameters and boundary
conditions. Theoretical “corrections” to
measured heat flow are thus uncertain.
Near-axis hydrothermal
cooling accounts for about 1 TW of the global heat flux.
The extent of hydrothermal cooling due to off-axis circulation
of cold water is usually taken as the difference between
the predictions of the plate cooling model and the observed
conducted heat flow but there is no theoretical basis
for this. The plate cooling model predicts higher heat
flows than observed out to ~ 50 Ma, and lower than observed
thereafter. Some of the differences between the measured
and theoretical heat flows are due to causes other than
hydrothermal circulation, including off-axis intrusions
and lateral variability in mantle potential temperature.
Hydrothermal
circulation
The mean oceanic
heat flux is sometimes determined by fitting the parameters
of a half-space or plate cooling model to bathymetry
and heat-flow data on older oceanic lithosphere. This
approach substantially overestimates the heat flux for
ages less than 40 My but the discrepancy persists out
to 60 My. The calculated Quaternary flux exceeds the
measurements by 500% (Hofmeister
& Criss,
2003). The mean calculated oceanic value (Pollack
et al., 1993) is about double the median observed
flux of 65 mW/m2 for the oceans and 61 mW/m2
for the continents. Theoretical cooling models generally
use a constant conductivity and ignore its temperature
dependence. In addition, the hydrothermal contribution
has been overestimated. According to Hofmeister
& Criss
(2003), both the theoretical heat flux values and
the hydrothermal contribution should be reduced. In
addition to the conducted heat, other processes which
affect the heat flow as a function of age include intrusion,
underplating, stress changes in the plate and serpentization,
which are problematic to assess. Table 1a includes estimates
of near-ridge hydrothermal circulation but otherwise
tabulates the measured conducted heat flow values.
Expected
background variations in heat flow
Boundary-layer
and plate models attribute all variations in bathymetry
and heat flow to conductive cooling as a function of
time. However, mantle convection and plate tectonics
could not exist, and are inconsistent, with an isothermal
mantle. Lateral temperature variations of the mantle
below the plate of at least ± 100°C are expected.
For a 100-km-thick thermal boundary layer this implies
heat flow variations of about ±15% superposed on normal
cooling curves. Variations in permeability at the top
of the plate cause variations in the hydrothermal component
of heat flow, and this component of heat flow must be
allowed for separately. The important point here is
that temporal changes in surface heat flux must be considered
before concluding that an “energy crisis”
exists.
Global heat flow variations can be estimated
using seafloor age reconstructions [Reference list
D; Loyd
et al. (2007) and references therein]. Loyd
et al. (2007)
show that heat flow has decreased by 0.15% every million
years during the Cenozoic due to a decrease in the
area of ridge-proximal oceanic crust. This is an order
of magnitude faster than estimates based on smooth,
parameterized cooling models. This implies that heat
flow experiences short-term fluctuations associated
with plate tectonic cyclicity.
The cooling plate model assumes that the mantle
beneath the plates is isothermal and homogeneous and
has the same potential temperature as midocean ridges
basalts. Tomography shows that the subplate mantle is
heterogeneous.
|
Heat
sources
Radioactivity
All estimates
of terrestrial abundances of the heat-producing elements
depend on meteorite compositions (Reference List C).
Carbonaceous chondrites are the usual choice, but enstatite
achondrites and meteorite mixes are also used. The Earth
is unlikely to match any given class of meteorite since
it condensed and accreted over a range of temperatures
from a range of starting materials. The refractory elements
are likely to occur in the Earth in cosmic ratios, but
the volatile elements are depleted. The large metallic
core indicates that the Earth, as a whole, is a reduced
body, although at least the crust and the outer shells
of the mantle are oxidized. Enstatite achondrites match
the Earth in the amount of reduced iron (oxidation state)
and in oxygen isotopic composition and have been used
to estimate terrestrial abundances.
Estimates for
the heating potential of the Bulk Silicate Earth (BSE
= crust + mantle) range from 12.7 to 31 TW, although
most authors obtain values in a much more restricted
range (Table 1b). These are present-day instantaneous
values. Heat conducted through the surface was generated
some time ago, when the radioactive abundances were
higher, so estimates of radioactive heating, based on
current radioactive contents, are lower bounds on the
contribution of radioactive elements to the present-day
surface heat flow, assuming that the estimates of U,
Th, and K are realistic. The allowable variation in
U and Th contents of the mantle is a large fraction
of the postulated discrepancy between production and
heat flow. Production of heat can be much larger if
K contents have been underestimated. It is of interest
that, because of the short half-life of 40K,
most of the 40Ar in the atmosphere was generated
in early Earth history. More efficient degassing then
may partly explain the large fraction of the terrestrial
40Ar that is in the atmosphere.
The amount of
radioactivity in the crust must be subtracted out in
order to obtain mantle abundances and heat productivities.
Using 8 TW as the best estimate of crustal productivity
gives < 23 TW as the current energy output from mantle
radioactivity. Heat from the core (about 9 TW), solid
Earth tides (1 to 2 TW) and thermal contraction (~ 2
TW) are non-radiogenic sources that may add 12-13 TW
to the mantle heat flow, about the same as the current
(non-delayed) mantle radiogenic contribution. The radiogenic
contribution can be increased by about 25% if it takes
1 Gyr to reach the base of the lithosphere. On top of
all this is secular cooling of the mantle. In a chemically
stratified mantle, the outer layers cool much faster
than the deeper layers. If cooling is confined to the
upper 1,000 km a temperature drop of 50 K/Ga corresponds
to a heat flow of 3 TW. Cooling rates of twice this
value may be plausible (Reference List D).
There thus appears
to be no need for any exotic heat sources or hidden
sources of radioactivity in the mantle. This conclusion
is independent of the uncertain contribution of hydrothermal
circulation to the surface heat flow. There are implications,
however, for the temperatures of the Archean mantle
and the style of convection, and the mechanisms of heat
removal (Reference List D). The present styles of mantle
convection and plate tectonics are unlikely to have
operated in the Archean (Hamilton, 2003). |
Table
1: a) Measurements and estimates of global heat flow.
Some of these are from a recent review by Hofmeister
& Criss
(2003) and some are from standard sources (e.g.,
AGU Handbooks). b) Sources of thermal energy in the
Earth's interior (from Reference Lists A, C & E).
a)
Heat flow
|
TW
|
|
|
Average
of world-wide measurements |
30-32
|
“Corrected”
for ridge effect |
43
|
Cooling
halfspace model |
41-44
|
|
|
Near-ridge
hydrothermal |
1
|
Temporal
variations |
±
2
|
b)
Energetics
|
TW
|
|
|
Potential
energy contributions |
|
Conducted from
core |
8.6 |
Mantle
differentiation |
0.6
|
Thermal
contraction |
2.1
|
Earthquake
induced gravitational energy |
2
|
Radiated
seismic energy |
0.3
|
Tidal
friction |
1-2
|
Total
(possible non-radiogenic and core sources) |
~16
|
|
|
Radiogenic |
|
Radiogenic
(BSE) |
19
- 31
|
Radiogenic
(continental crust) |
5.8-8
|
Delayed
radiogenic
(1 to 2 Ga delay between production & arrival
at surface) |
5
|
Total
(radiogenic) |
24-36
|
|
|
Secular
cooling (Korenaga, 2003; Schubert
et al., 1980) |
|
80
K/Ga |
9
|
100
K/Ga |
14
|
|
|
Total
input |
39-66
|
Slab melting
It is often assumed
that the melting involved in hotspot magmatism requires
an anomalous source of energy, e.g., plumes or
frictional heating. Heat is indeed required, and invoking
a source with a lower melting point e.g., eclogite,
does not remove this problem since latent heat of melting
is still required.
The mantle is
a much larger source of energy than the core. Melting
can occur by placing material with a low melting point
(e.g., basalt, eclogite, pyroxenite, piclogite)
into the shallow mantle by subduction or delamination
of continental lithosphere. The surrounding mantle serves
as a heat source and the subducted/delaminated material
as a heat sink. At thermal equilibrium the slabs, at
least their upper portions, will be partially molten,
except in the coldest parts of the shallow mantle. If
the slab is rich in volatiles (H2O, CO2)
this will reduce seismic velocity, even though the slab
is cooler than the surrounding mantle. Subduction refertilizes
the mantle, cools it, and causes major local thermal
perturbations.
Some of the seismically
slowest regions on Earth – low velocity zones
(LVZ) – are at the tops of and above subducting
slabs (mantle wedge, back-arc basins). These regions
are cooled by subduction. The LVZ extend to depths of
200-300 km. Presumably if such convergent regions turn
into diverging regions, such as when old sutures are
reactivated during continental breakup, the fertile,
volatile-rich regions will melt and may even provide
dense sinkers which liberate volatiles as they sink
(see Lithospheric Delamination
page) giving deep LVZ and basaltic melts on top.
A sinking carbonated eclogitic slab can have low seismic
velocities!
Slabs
and delaminated eclogite accumulating at say 650
km can be entrained into upwelling flows when continents
diverge and can be part of the basaltic source material.
Thus, slabs do not need to be carried around by continents
in order to explain slab components in hotspot magmas,
although some slab material will certainly be trapped
between suturing Archaean cratons. The largest divergence
suction is expected with thick separating cratons,
whereas thin diverging lithosphere will suck up mainly
shallow material. Material in the transition region
can also be displaced by sinking slabs. |
Summary
The heat budget
of the Earth cannot be treated as an instantaneous one-dimensional
heat flow problem, or one that involves a homogeneous
mantle with uniform and static boundary conditions.
Both the radial and lateral structure of the Earth must
be considered. Continents affect mantle heat flow by
diverting heat to the ocean basins (Lenardic,
1998) and drifting so as to be over cold downwellings
(Reference List E). Chemical stratification (Reference
List B) of the mantle slows down cooling of the Earth
but the upward concentration of radioactive elements
reduces the time between heat generation and surface
heat flow. Nevertheless, one-dimensional and homogeneous
models, or models with a downward increase in radioactive
heating have dominated the attention of convection modelers
(Reference List D). Paradoxes such as the “missing
heat source problem” can be traced to non-realistic
assumptions and initial and boundary conditions.
The major outstanding
problems in the Earth's thermal budget and history involve
the role of hydrothermal circulation near the top, and
radiative heat transfer near the bottom, of the mantle.
Convection modeling has not yet covered the parameter
range which may be most pertinent from physical considerations
and geophysical data. What is needed is a thermodynamically
self-consistent approach which includes the temperature,
pressure and volume-dependence of physical properties,
realistic initial and boundary conditions, and the ability
to model melting and various forms of heat transport.
The bottom line
is that there appears to be no mismatch between observed
heat flow and plausible sources of heating. The rate
at which the mantle is losing heat appears to have been
overestimated in the past, and the available energy
sources in the interior have been underestimated. The
uncertainties in the various estimates have not been
fully appreciated (see Hofmeister
and Criss,
2003, for a discussion). Lord Kelvin also underestimated
the errors in his estimates of the age of the Earth,
and neglected, understandably, a significant heat source. |
Bibliography
A.
Heat flow compilations
-
-
Jaupart, C. & Mareschal, J.-C.,
2003, Treatise on Geochemistry, in Heinrich
D. Holland & Karl K. Turekian, Eds, Constraints
on Crustal Heat Production from Heat Flow Data,
Elsevier.
-
Jaupart, C. & J.-C. Mareschal,
1999, The thermal structure of continental roots,
Lithos, 48, 93-114.
-
Nyblade, A.A., & Pollack,
H.N., 1993, A global analysis of heat flow from
Precambrian terrains: implications for the thermal
structure of Archean and Proterozoic lithosphere,
J. Geophys. Res., 98,
12,207-12,218.
-
O'Connell, R. J. & B. H. Hager,
1980, On the thermal state of the Earth, in
A. Dziewonski & E. Boschi, Eds., Physics
of the Earth's Interior, Proc. Enrico Fermi
Int. Sch. Phys., 78, 270-317.
-
Pollack, H.N., Hurter, S.J., &
Johnson, J.R., 1993, Heat loss from the Earth's
interior: analysis of the global data set, Rev.
Geophys., 31, 267-280.
-
Pollack, H. N., 1982, The heat
flow from the continents, Ann. Rev. Earth
Planet. Sci., 10, 459-481.
-
Sclater, J. G., Jaupart, C. &
Galson, D., , The heat flow through oceanic
and continental crust and the heat loss
of the Earth, Rev. Geophys. Space Phys., 18,
269-312.
-
Stein, C., & D. Abbott, 1991,
Heat-flow constraints on the south-Pacific superswell,
J. Geophys. Res., 96,
16,083-16,100.
-
Stein, C.A., & S. Stein,
1992, A model for the global variation in
oceanic depth and heat-flow with lithospheric
age, Nature,
359, 123-129.
-
Stein, C.A., & S. Stein,
1993, Constraints on Pacific midplate swells
from global depth-age and heat flow-age models,
in The Mesozoic
Pacific: Geology, Tectonics, and Volcanism,
53-76, American Geophysical Union, Washington,
D.C..
-
von Herzen, R.P., M.J. Cordery,
R.S. Detrick, & C. Fang, 1989, Heat-flow
and the thermal origin of hot spot swells -
the Hawaiian swell revisited, J. Geophys.
Res., 94, 13,783-13,799.
-
Vitorello,
I., & Pollack, H. N., 1980, On the variation
of continental heat flow with age and the
thermal evolution of continents, J. Geophys.
Res., 85, 983-995.
B. Chemical stratification
and distribution of radioactive elements
-
Agee, C. B., & D. Walker,
1993, Olivine flotation in mantle melt, Earth
Plant. Sci. Lett., 114,
315-324.
-
Agee, C. B. & Walker, D.,
1988, Mass balance and phase density constraints
on early differentiation of chondritic mantle,
Earth Planet. Sci. Lett., 90,
144.
-
Anderson, D.L., 1989, Theory
of the Earth, Blackwell Scientific Publications,
Boston, 366 pp. http://resolver.caltech.edu/CaltechBOOK:1989.001
-
Anderson, D. L., 1989, Where on
Earth is the Crust?, Physics Today, 42,
38-46.
-
Anderson, D L , 2002, The Case
for Irreversible Chemical Stratification of
the Mantle, Int. Geol. Rev., 44,
97-116.
-
Anderson, D.L. 2005. Self-gravity,
self-consistency, and self-organization in
geodynamics and geochemistry, in Earth's
Deep Mantle: Structure, Composition, and Evolution,
Eds. R.D. van der Hilst, J. Bass, J. Matas & J.
Trampert, AGU Geophysical Monograph Series
160, pp. 165-186.
-
Anderson, D.L., 2005, Scoring
hotspots: The plume and plate paradigms, in
Foulger, G.R., Natland, J.H., Presnall, D.C.,
and Anderson, D.L., eds., Plates,
plumes, and paradigms: Geological Society
of America Special Paper 388, 31-54.
-
Anderson,
D.L., 2006, Speculations on the nature and
cause of mantle heterogeneity, Tectonophysics, 416,
7-22.
-
Boschi, L. & Dziewonski,
A. M., 1999, “High” and “low”
resolution images of the Earth's mantle - Implications
of different approaches to tomographic modeling,
J. Geophys. Res., 104,
25,567-25,594.
-
Clark, S. P., & Turekian,
K. K., 1979, Thermal constraints on the distribution
of long-lived radioactive elements in the Earth,
Phil. Trans. R.Soc. Lond., 291,
269-275.
-
Cousens, B.L. et al., 2001, Enriched
Archean lithospheric mantle beneath western
Churchill Province, Geology, 29,
827-830.
-
Cserepes, L., Yuen, D. A., &
Schroeder, B. A., 2000, Effect of the mid-mantle
viscosity and phase-transition structure of
3D mantle convection, Phys. Earth. Planet.
Int., 118, 135-148.
-
Davaille, A., 1999, Two-layer
thermal convection in miscible viscous fluids.
J. Fluid Mech., 379,
223-253.
-
Davies, G. F., 2000, Dynamic
Earth: Plates, Plumes and Mantle Convection,
Cambridge University Press, Cambridge, 458 pp.
-
Fukao Y, Obayashi M, Inoue H,
Nenbai M., 1992, Subducting slabs stagnant in
the mantle transition zone, J. Geophys. Res.,
97, 4809-4822.
-
Fukao, Y., Widiyantoro, S., Obayashi,
M., 2001, Stagnant slabs in the upper and lower
mantle transition region, Rev. Geophy.,
28, 291-323.
-
Gasparik, T., 1993, The role of
volatiles in the transition zone, J. Geophys.
Res., 98, 4287-4299.
-
Gasparik, T., 1997, A model for
the layered upper mantle, Phys. Earth Planet.
Int., 100, 197-212.
-
Wen, Lianxing & D. L. Anderson,
1995, The Fate of Slabs Inferred from Seismic
Tomography and 130 Million Years of Subduction,
Earth Planet Sci. Lett., 133,
185-198.
-
Wen, L. & D. L. Anderson,
1997, Layered mantle convection: A model for
geoid and topography, Earth Planet. Sci.
Lett., 146, 367-377.
C. Energy sources in the Earth
-
Birch, F., 1965, Energetics of
core formation, J. Geophys. Res., 70,
6217-6221.
-
Chao, B.F., Gross, R. & Dong,
D.-N., 1995, Changes in global gravitational
energy induced by earthquakes, Geophys. J.
Int., 122, 784-789
-
Flasar, F. M. & Birch, F.,
1973, Energetics of core formation: a correction.
J. Geophys. Res., 78,
6101-6103.
-
Gubbins, D., 1977, Energetics
of the Earth's core, J. Geophys., 43,
453-464.
-
Gubbins, D., Masters, T.G. &
Jacobs, J.A., 1979. Thermal evolution of the
Earth's core, Geophys. J. R. astr. Soc.,
59, 57-99.
-
Javoy, M., 1999, Chemical Earth
models, C. R. Acad. Sci. Paris, 329,
537-555.
-
Jochum, K. P., Hofmann, A. W.,
Ito, E., Seufert, H. M. & W.M. White, 1986,
K, U and Th in mid-ocean ridge basalt glasses
and heat production, K/U and K/Rb in the mantle,
Nature, 306, 431-436.
-
McDonough, W. F., 1995, The composition
of the Earth, Chem. Geol., 120,
1535-1540.
-
Rudnick, R. L., McDonough, W.
F. & O'Connell, R. J., 1998, Thermal structure,
thickness and composition of continental lithosphere.
Chem. Geol., 145, 395-411.
-
Stacey, F.D. & Stacey, C.H.B.,
1999, Gravitational energy of core evolution:
implications for thermal history and geodynamo
power, Phys. Earth Planet. Inter., 110,
83-93.
-
Van Schmus W.R., 1995, Natural
radioactivity of the crust and mantle, in A
Handbook of physical constants, AGU
References shelf 1. Ed. T.J. Ahrens, AGU, Washington
DC, pp. 283-291.
-
Verhoogen, J., 1980, Energetics
of the Earth, Nat. Acad. Sci., Washington,
DC, 139 pp.
-
White, W.M. 1983, K, U and Th
in mid-ocean ridge basalt glasses and heat
production. K/U and K/Rb in themantle. Nature, 306,
431-436.
-
Anderson, D.L., 2007, The Eclogite
engine: Chemical geodynamics as a Galileo thermometer.
In: Foulger, G. R. & Jurdy, D. M. (eds.)
Plates, Plumes and Planetary
Processes. Geological
Society of America, Special Paper 430, 47–64.
-
Christensen, U., 1985, Thermal
evolution models for the Earth, J. Geophys.
Res., 90, 2995-3007.
-
Coltice, N., & Ricard, Y.,
1999, Geochemical observations and one layer
mantle convection, Earth Planet. Sci. Lett.,
174, 125-137.
-
Conrad, C. P., & B. H. Hager,
2001, Mantle convection with strong subduction
zones, Geophys. J. Int., 144,
271-288.
- Kaula, W.M., 1983, Minimal upper mantle temperature
variations consistent with observed heat flow and
plate velocities, J. Geophys.
Res., 88, 10,323-10,332.
-
Korenaga, J., 2003, Energetics
of mantle convection and the fate of fossil
heat, Geophys. Res. Lett., 30,
1437, doi:10.1029/2002GL016179.
-
Korenaga, J., Urey
ratio and the structure and evolution of Earth's
mantle, Rev.
Geophys., 46, RG2007,
doi:10.1029/2007RG000241, 2008.
-
Korenaga, J., 2008, Comment on "Intermittent
plate tectonics?, Science, 320,
1291a.
-
Loyd, S. J., T. W. Becker, C.
P. Conrad, C. Lithgow-Bertelloni & F. A.
Corsetti, 2007, Time-variability in Cenozoic
reconstructions of mantle heat flow: Plate
tectonic cycles and implications for Earth’s
thermal evolution, Proceedings
of the National Academy of Science, U.S.A., 104,
14,266–14,271.
-
McNamara, A.K., & P.E. van
Keken, P.E., 2000, Cooling of the Earth: A parameterized
convection study of whole versus layered models,
Geochemistry, Geophysics, Geosystems,
1, 183-190.
-
Tozer, D. C., 1972, The present
thermal state of the terrestrial planets, Phys.
Earth Planet. Inter., 6,
182-197.
-
Schubert, G., Stevenson, D. &
Cassen, P., 1980, Whole planet cooling and the
radiogenic heat source contents of the Earth
and Moon, J. Geophys. Res., 85,
2531-2538.
-
Schubert, G., Turcotte, D., Olson,
P., 2001, Mantle convection in the Earth
and planets, C. U. Press, 956 pp.
-
Stacey, F. D., 1992, Physics
of the Earth, 2nd Ed. Brisbane, Brookfield
Press.
-
Stacey, F. D. & Loper, D.
E., 1984, Thermal histories of the core and
mantle, Phys. Earth Planet. Inter., 36,
99-115.
-
Stevenson, D., Spohn, T. &
Schubert, G., 1983, Magnetism and thermal evolution
of the terrestrial planets, Icarus, 54,
466-489.
-
Tackley, P., 1998, Three dimensional
simulations of mantle convection with a thermo-chemical
basal boundary layer: in: M. Gurnis, M. et al.,
eds., The Core-Mantle Boundary Region,
Washington, AGU, 334 pp.
-
Thompson, Sir W. (Lord Kelvin),
1890, On the Secular cooling of the Earth.
Mathematical and Physical Papers, Vol III,
Elasticity, Heat, Electro-Magnetism. London:
C.J. Clay and sons, pp. 295-311.
-
Van Keken PE, Ballentine C.J.,
1998, Whole-mantle versus layered mantle convection
and the role of a high-viscosity lower mantle
in terrestrial volatile evolution, Earth
Planet. Sci. Lett., 156,
19-32.
-
Van Keken P.E., Ballentine C.J.,
1999,. Dynamical models of mantle volatile
evolution and the role of phase transitions
and temperature-dependent rheology, J.
Geophys. Res., 104,
7137-51.
-
Guillou, L. & Jaupart, C.,
1995, On the effect of continents on mantle
convection, J. Geophys. Res., 100,
24,217-24,238.
-
Lenardic, A., & L.-N. Moresi,
2001, Heat flux scalings for mantle convection
below a conducting lid: resolving seemingly
inconsistent modeling results regarding continental
heat flow, Geophys. Res. Lett., 28,
1311-1314.
-
Lenardic, A., L. Guillou-Frottier,
J.-C. Mareschal, C. Jaupart, L.-N. Moresi, &
W.M. Kaula, 2000, What the mantle sees: the
effects of continents on mantle heat flow, In,
The History and Dynamics of Global Plate
Motions, Ed: M. Richards, R. Gordon, &
R. van der Hilst, AGU Press, 95-112.
-
Lenardic, A., 1998, On the partitioning
of mantle heat loss below oceans and continents
over time and its relationship to the Archean
paradox, Geophys. J. Int., 134,
706-720.
-
Crough, S.T., 1983, Hotspot swells,
Ann. Rev. Earth Planet. Sci., 11,
165-193.
-
McNutt, M. K. & A. V. Judge,
1990, The Superswell and mantle dynamics beneath
the South Pacific, Science, 248,
969-975.
-
Parsons, B. & J. G.
Sclater, 1977, An analysis of the variation
of the ocean floor bathymetry and heat flow
with age, J. Geophys. Res., 82,
803-827.
- Phipps Morgan, J., W. J. Morgan, and E. Price,
1995, Hot spot melting generates both hot spot
volcanism and a hot spot swell? J. Geophys.
Res., 100, 8045-8062.
-
Phipps Morgan, J. & W. H.
F. Smith, 1992, Flattening of the seafloor
depth-age curve as a response to asthenospheric
flow,
Nature, 359, 524-527.
-
Phipps Morgan, J. & W. H.
F. Smith, 1994, Correction: Flattening of the
seafloor depth-age curve as a response to asthenospheric
flow, Nature, 371, 83.
-
Rowley, D.B., 2002, Rate of plate
creation and destruction: 180 Ma to Present,
Geol. Soc. Am. Bull., 114,
927-933.
-
Sandwell, D. T., E. L. Winterer,
J. Mammerickx, R. A. Duncan, M. A. Lynch, D.
A. Levitt, and C. L. Johnson, 1995, Evidence
for diffuse extension of the Pacific plate
from the Pukapuka Ridges and crossgrain gravity
lineations, J. Geophys.
Res., 100, 15087-15099.
-
Sleep,
N.H., 1994, Lithospheric thinning by midplate
mantle plumes and the thermal history of hot
plume material ponded at sublithospheric depths, J. Geophys. Res., 99,
9327-9343.
-
Smith, W. H. F. & J. Phipps
Morgan, 1992, A dynamic origin for asymmetric
subsidence and geoid anomalies in the south
Atlantic Ocean? Eos, Trans. Am. Geophys.
Union, 73, 582.
-
Stein, C. A. & S. Stein, 1994,
Comparison of plate and asthenospheric flow
models for the thermal evolution of oceanic
lithosphere, Geophys. Res. Lett., 21,
709-712.
|
last
updated 15th November, 2009 |
|
|