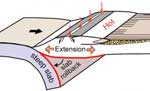 |
Geochemical transfer from the subducting crust into the mantle |
Yong-Fei Zheng
CAS Key Laboratory of Crust-Mantle Materials and Environments, School of Earth and Space Sciences, University of Science and Technology of China, Hefei 230026, China; yfzheng@ustc.edu.cn
This webpage is a summary of: Zheng, Y.-F., 2019. Subduction zone geochemistry. Geoscience Frontiers, 10, 1223-1254.
How were geochemically enriched signatures transferred from the lithosphere into the magma source of oceanic basalts? The answer would come quickly from people who work on the origin of island arc basalts (IAB) in interplate regions: they were carried by subducting crust-derived liquid phases such as water and hydrous melts. However, no clear answer would be given by people who work on ocean island basalts (OIB) in regions that are presently intraplate. Now this issue has been highlighted by Zheng (2019) from inspection of subduction zone geochemistry.
Geochemically, there are two kinds of enrichment in oceanic basalts relative to primitive mantle. The first is the enrichment in radiogenic isotope composition, with high (87Sr/86Sr)i and negative εNd(t) values. The second is enrichment in melt-mobile trace elements such as large ion lithophile elements (LILE) and light rare earth elements (LREE) (Figure 1). These two kinds of geochemical enrichment occur not only in IAB and OIB but also in mid-ocean ridge basalts (MORB) despite their compositional variation from normal through transitional to enriched. Enriched MORB has similar compositions to common OIB.
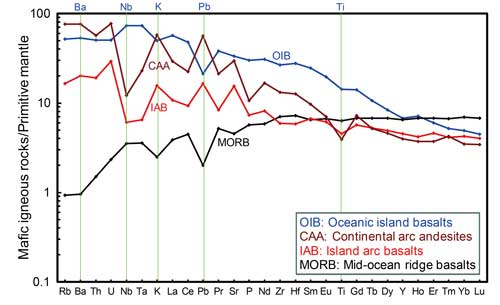
Figure 1: Trace element distribution of mafic volcanics in the primitive mantle-normalized diagram (revised after Zheng, 2012).
Although the two kinds of geochemical enrichment in IAB are uncontroversially attributed to crustal recycling by subduction, those in OIB are explained in different ways. Whereas the enrichment of radiogenic Sr-Nd isotopes in OIB is also ascribed to crustal recycling, the enrichment of LILE and LREE in OIB is explained by assuming a source in the lower mantle that was sampled by mantle plumes originating from the core-mantle boundary (Hofmann & White, 1982; Allègre & Turcotte, 1986; Zindler & Hart, 1986). While this model has been popular for the past four decades (Hofmann, 1997), it is often questioned by people who work on subduction zone geochemistry.
There are two important differences in trace element composition between IAB and OIB. The first is the abundance of water-soluble trace elements such as Pb (Figure 1). Typically, IAB are characterized by enrichment in Pb, whereas OIB are characterized by depletion in Pb. How can this be explained? It must originate in the dehydration behavior of crustal rocks at subarc to postarc depths. Because Pb is highly soluble in water (Brenan et al., 1995b; Kogiso et al., 1997; Kessel et al., 2005), metamorphic dehydration at subarc depths leads to significant loss from subducting oceanic crust. As a result, Pb is readily depleted in deeply subducting crust at postarc depths.
The second difference is the abundances of Nb and Ta (Figure 1). Typically, IAB are depleted in Nb and Ta whereas OIB are enriched. Together with the difference in the Pb abundances, this leads to much lower Nb/U and Ce/Pb ratios for IAB than OIB and MORB (Hofmann et al., 1986; Miller et al., 1994). It is known that HFSE such as Nb, Ta, Zr and Hf are preferentially partitioned into rutile (Foley et al., 2000), and that rutile has very low solubilities in subduction zone fluids (e.g., Brenan et al., 1994, 1995a). For these reasons, the depletion of Nb and Ta in IAB is attributed to the stability of rutile in subducting oceanic crust at subarc depths.
The experimental study of Ryerson & Watson (1987) indicates that rutile can become more soluble in hydrous felsic melts at elevated temperatures. In combination with the fractionation between melt-mobile and -immobile trace elements during dehydration melting of crustal rocks, Ringwood (1990) proposed that OIB-like geochemical signatures in oceanic basalts can be produced by partial melting of deeply subducted oceanic crust with rutile breakdown at postarc depths (Figure 2). However, this proposal was overlooked in the last century because of lack of knowledge of the solubility of rutile in hydrous melts. It has now been revisited by Zheng (2019) after examining new experimental data on rutile solubility in hydrous felsic melts.
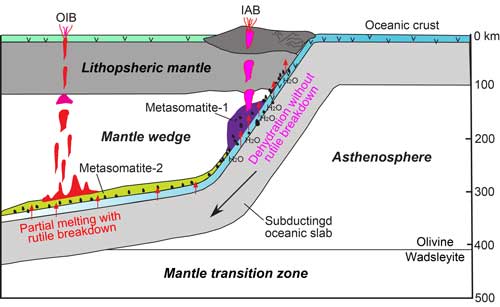
Figure 2: Schematic diagram showing formation of a mantle wedge during subduction of an oceanic plate (adapted from Ringwood, 1990). The mantle wedge increases in thickness with distance from the trench. It is relatively thin where island arc basalts (IAB) are sourced but thick where ocean island basalts (OIB) are sourced. As a result dehydration and melting of the subducting oceanic crust at different depths produce different liquid-phase compositions. Metasomatite-1 denotes serpentinized to chloritized peridotites at subarc depths, and metasomatite-2 denotes pyroxenite to hornblendite at postarc depths.
These observations and interpretations lead to the conclusion that the differences in the compositions of IAB and OIB are primarily determined by the depths of dehydration and melting in subducting slabs. This is, in turn, dictated by the thermal regime of subduction zones. Petrological studies of high-pressure to ultra-high-pressure eclogite facies metamorphic rocks from both oceanic and continental subduction zones indicate that lithospheric subduction generally proceeds not only at low angles but also at low thermal gradients (<10-15°C/km) in the early stage. As a consequence, there is only significant dehydration of the subducting crust at lithospheric depths, with very limited melting in the rutile stability field. Because the lowest temperatures were kept at the slab-mantle interface, the mantle wedge was significantly cooled during low-angle subduction. Thus, its center did not maintain high temperatures of 1000-1200°C in this stage. Under these conditions the mantle wedge cannot be partially melted by fluxing of liquid phases at subarc depths. Instead, the mantle wedge would have been metasomatized by liquid phases and enriched in fluid-mobile trace elements and their pertinent radiogenic isotopes.
Continued subduction would have led to slab rollback when the rate of gravitational sinking exceeds the rate of plate convergence. In this case, the asthenospheric mantle can flow laterally into the space between the mantle wedge and the descending slab. As a result, the mantle wedge base may be heated by the asthenospheric mantle leading to partial melting of metasomatic domains (Metasomatite-1 in Figure 2). In this model, mafic arc magmatism at convergent plate boundaries proceeds via at least in two stages (Figure 3), the first of which is source formation and the second which is source melting.
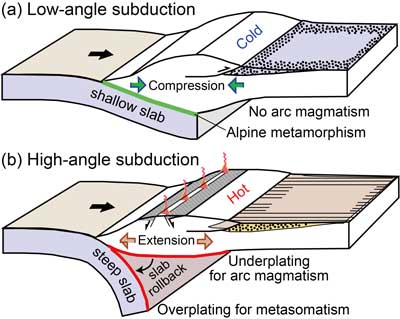
Figure 3: Schematic diagram showing tectonic evolution during subduction of an oceanic slab (abstracted from Zheng, 2019). The oceanic slab subducts at a low angle in the early stage but rolls back at a high angle later. Underplating denotes partial melting of mafic to ultramafic metasomatites in the mantle wedge for mafic arc magmatism due to heating by the laterally filled asthenospheric mantle. Overplating denotes partial melting of crustal rocks on the rolling-back slab surface, due to heating by the asthenospheric mantle, producing ultramafic metasomatites.
If the slab is continuously subducted to post-arc depths after rollback, previously dehydrated crust undergoes partial melting with rutile breakdown at temperatures as high as 1300-1600°C. This produces felsic melts that are much less hydrous and depleted in Pb but enriched in Nb and Ta. Such melts react with the mantle wedge peridotite of asthenospheric origin generating fertile enriched domains (Metasomatite-2 in Figure 2) which are the source of OIB. As soon as the metasomatites are partially melted at a later time, basalts with OIB-like geochemical signatures may be produced at the surface. Thus, OIB is also produced in a two-stage process.
In conclusion, OIB can be produced by metasomatite melting in the upper mantle and there is no need to hypothesize a lower mantle origin. In this framework, the behavior of trace elements in crustal rocks at different depths in and around subduction zones is the key to explaining geochemical transfer from the descending crust into the mantle.
References
-
Allègre, C.J., Turcotte, D.L., 1986. Implications of a two component marble-cake mantle. Nature 323, 123–127.
-
Brenan, J.M., Shaw, H.F., Phinney, D.L., Ryerson, F.J., 1994. Rutile-aqueous fluid partitioning of Nb, Ta, Hf, Zr, U, and Th: implications for high field strength element depletions in island-arc basalts. Earth and Planetary Science Letters 128, 327–339.
-
Brenan, J. M., Shaw, H. F., Ryerson, F. J., Phinney, D. L., 1995a. Mineral-aqueous fluid partitioning of trace elements at 900°C and 2.0 GPa: constraints on the trace element chemistry of mantle and deep crustal fluids. Geochimica et Cosmochimica Acta 59, 3331-3350.
-
Brenan, J.M., Shaw, H.F., Ryerson, R.J., 1995b. Experimental evidence for the origin of lead enrichment in convergent margin magmas. Nature 378, 54–56.
-
Foley, S.F., Barth, M.G., Jenner, G.A., 2000. Rutile/melt partition coefficients for trace elements and an assessment of the influence of rutile on the trace element characteristics of subduction zone magmas. Geochimica et Cosmochimica Acta 64, 933–938.
-
Hofmann, A.W., White, W.M., 1982. Mantle plumes from ancient oceanic crust. Earth and Planetary Science Letters 57, 421–436.
-
Hofmann, A.W., Jochum, K.P., Seufert, M., White, W.M., 1986. Nb and Pb in oceanic basalts: new constraints on mantle evolution. Earth and Planetary Science Letters 79, 33-45.
-
Hofmann, A.W., 1997. Mantle geochemistry: The message from oceanic volcanism. Nature 385, 219-229.
-
Kessel, R., Schmidt, M.W., Ulmer, P., Pettke T., 2005. Trace element signature of subduction-zone fluids, melts and supercritical liquids at 120-180 km depth. Nature 437, 724-727.
-
Kogiso, T., Tatsumi, Y., Nakano, S., 1997. Trace element transport during dehydration processed in the subduction oceanic crust: 1. Experiments and implications for the origin of ocean island basalts. Earth and Planetary Science Letters 148, 193-205.
-
Miller, D.M., Goldstein, S.L., Langmuir, C.H., 1994. Cerium/lead and lead isotope ratios in arc magmas and the enrichment of lead in the continents. Nature 368, 514-520.
-
Ringwood, A.E., 1990. Slab-mantle interactions: 3. Petrogenesis of intraplate magmas and structure of the upper mantle. Chemical Geology 82, 187-207.
-
Ryerson, F.J., Watson, E.B., 1987. Rutile saturation in magmas: implications for Ti–Nb–Ta depletion in island-arc basalts. Earth and Planetary Science Letters 86, 225–239.
-
Zheng, Y.-F., 2012. Metamorphic chemical geodynamics in continental subduction zones. Chemical Geology 328, 5-48.
-
Zheng, Y.-F., 2019. Subduction zone geochemistry. Geoscience Frontiers 10, 1223-1254.
-
Zindler, A., Hart, S., 1986. Chemical geodynamics. Annual Review of Earth and Planetary Sciences 14, 493-571.
last updated 8th
June, 2019 |