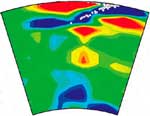 |
Do
low-Ti basalts require a subcontinental lithospheric
mantle source? |
Alexei V. Ivanov
Institute
of the Earth’s Crust, Siberian Branch, Russian
Academy of Sciences, Irkutsk, Russia
aivanov@crust.irk.ru
Postulate:
low-Ti basalts = subcontinental lithospheric
mantle source.
Is this right?
|
In their recent
contribution on Karoo, Jourdan et al. (2007) write
that subcontinental lithospheric mantle (SCLM) is required
for origin of the Karoo flood basalts. As proof of
this they suggest negative high-field-strength element
(HFSE) anomalies and a “wet” mantle source,
shown by biotite in some intrusive rocks, amongst other
evidence. A negative HFSE anomaly is a classic subduction
fingerprint, however. A “wet” source
is also a characteristic of a subduction environment,
so, why do Jourdan
et al. (2007) conclude that these two subduction
signatures indicate SCLM?
Attribution of subduction geochemical
fingerprints to SCLM is a conventional interpretation
that started in the 1980s (e.g., Hawkesworth
et al., 1984).
It is based on the logic that volcanism far away (say
more than a few hundred kilometers) from convergent
plate boundaries cannot be related to subduction and
thus subduction-like geochemistry must be attributed
to SCLM previously modified by paleosubduction.
This interpretation is based on the outdated
view of water transport into the mantle by subducting
slabs. In the 1980s it was thought that all subduction-related
water is released beneath volcanic arcs and none passes
into the deeper mantle. It is known today that such
a view is incorrect. Experimental discoveries of hydrous
phases and evidence for high water content in nominally
anhydrous minerals, together with numerical modeling,
show that at high rates of subduction significant amounts
of water can subduct as deep as the transition zone
and probably deeper (e.g., Litasov & Ohtani,
2003; Smyth et al., 2006; Komabayashi,
2006; Arcay et al., 2007; see also the AGU
monograph Earth’s
deep water cycle).
At ultracold subduction zones water can go into the
deep mantle even as high-pressure ice (Bina & Navrotsy,
2000)! Modeling (Bina et al., 2001) and seismic
observations (e.g., Song et al., 2004; Zhao
et al., 2004) show that some subducting slabs
can deflect to horizontal (stagnate by depth) and propagate
in this way far (thousands of kilometers) beneath continents.
Thus, there is a physical and natural explanation for
volcanism in the inner parts of continents, in particular
in back-arc tectonic settings in relation to active
(at the time of volcanism) subduction.
The paleotectonic setting
of Karoo
Consider the paleotectonic setting
of the Karoo flood basalt province (Figure 1). The
diameter of the province is about 2500 km. Its southern
(in present day coordinates) end was about 1500 km
from an active subduction trench in the Jurassic. Considering
prolonged Pantalassa (paleoPacific) subduction beneath
this part of Gondwana we can assume that the mantle
transition zone was full of slabs and therefore highly
hydrated (see paleotectonic reconstructions: Early
Carboniferous – Late
Carboniferous –
Late
Permian –
Early
Triassic –
Jurassic).
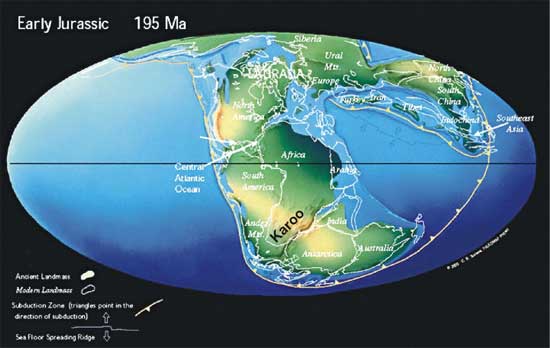
Figure 1. Paleotectonic reconstruction of Gondwana
in Early Jurassic time (from www.scotese.com).
The location of the Karoo flood basalt province is
added. It is located in area beneath which prolonged
subduction occurred (starting in the Carboniferous).
Subducting slabs probably stagnated and were trapped
in the mantle transition zone. Such slabs brought significant
amounts of water and dehydrated with time. Dehydration
led to volcanism similar in a sense to arc volcanism
but induced by much deeper dehydration.
Deep water cycle
Figure 2 shows experimental data on
water stability fields of mineral phases within mantle
peridotite and numerical calculations of slab buoyancy
(from Ivanov
et al., in press). This figure shows that fast
(cold) subducting slabs can bring significant amounts
of water into the deep mantle and that such slabs tend
to stagnate in the transition zone. With time such
slabs heat up to ambient mantle temperature and dehydrate.
Released water induces melting. If the released water
is not enough to induce melting because it is dissolved
in olivine (up to 0.9 wt.% of H2O can be
dissolved in olivine at the depth of the lowermost
upper mantle) it will lead to the volumetric expansion
of olivine (Smyth et al., 2006) and rise of
peridotitic diapirs (Ivanov et al., in press).
Such diapirs cross their solidus at ~ 250 km depth
(Figure 2). Low-Ti basalts with subduction trace-element
signatures in back-arc tectonic settings are likely
indicators of such process (Ivanov & Balyshev,
2005; Ivanov,
2007; Ivanov et al., in press).
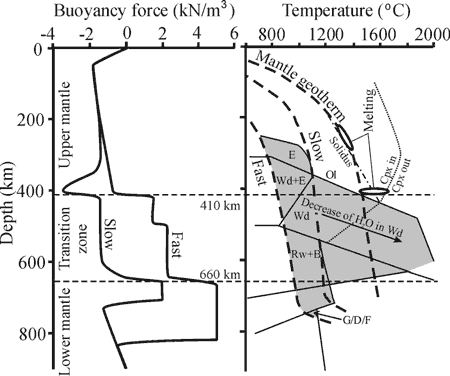
Figure 2. Saturation
of the transition zone with water via subduction and
possible regions of melting of water-saturated, advected
mantle peridotite (after Ivanov et al. in press). Left:
a fast subducting slab attains positive buoyancy while
crossing 410 km discontinuity. Both fast- and slow-subducting
slabs attain positive buoyancy while crossing 660 km
discontinuity (after Bina et al., 2001). Right:
stability fields (shadowed) of water-bearing minerals
in the CaO-MgO-Al2O3-SiO2-H2O
(CMAS+H2O) system (after Litasov & Ohtani,
2003). Water-bearing minerals: Wd – wadsleyite,
Rw – ringwoodite, E – dense hydrous magnesian
silicate, B and G/D/F – superhydrous phases.
Straight lines show boundaries between different mineral
assemblages (not included for the sake of simplicity).
Curved dotted line shows limits of clinopyroxene stability
field (cpx in and out). The symbol ‘Ol’ is
located in the region of highest solubility (up to
0.9 wt. %) of H2O by olivine (Smyth et al.,
2006). H2O solubility of olivine decreases
with increasing temperature (Smyth et al., 2006). White
ellipses mark possible depths of melting of water-saturated
mantle peridotite advected from the transition zone.
These regions are placed near or just above the solidus
line in the CMAS + 2 wt. % H2O system marked
by the hatch-dotted line (Litasov & Ohtani, 2003).
The depths of the regions of melting correspond well
to those suggested by Hirschman et al. (2005).
At intermediate depth between the two regions of melting
in the upper mantle is characterized by the highest
water capacity that prevents melting (Hirschman
et al., 2005).
Origin of the HFSE anomaly
Now consider why the subduction environment
is characterized by volcanic rocks with HFSE-negative
anomaly. It is almost universally agreed that the source
of melting beneath arcs (above subducting slabs) is
hydrated MORB mantle referred to as mantle wedge (e.g.,
Ulmer,
2001). Previously dry MORB-type mantle is hydrated
by water fluids derived from a subducting slab. Water
transports light ion lithophile elements (LILE), but
does not transport HFSE. Melting of such source enriched
by LILE yields melts enriched by LILE but not enriched
by HFSE. So, it is not HFSE-depletion, but LILE enrichment
that is responsible for the HFSE-depletion anomaly.
Does the depth of dehydration affect the magnitude
of the HFSE-depletion anomaly? Yes, because the activity
of H2O is dependent on temperature (Komabayashi,
2006) and deeper dehydration occurs at higher temperatures.
For deep dehydration (400-600 km) water fluid will
transport more HFSE compared to shallow (~100-150
km) dehydration. Thus, we should expect that magmatism
related to deep dehydration will be similar to island-arc
magmatism, but with less prominent subduction signatures
(e.g., a smaller HFSE-anomaly).
Some other examples of flood
basalts with low-Ti basalts
The Taos plateau in the northern part
of the Rio Grande rift (western US), which is the youngest
(about 4 Ma) flood basalt province on Earth with low-Ti
basalts, also referred to as high alumina olivine tholeiites
(HAOT), is about 1500 km away from a present-day convergent
boundary. Leat et al. (1988) suggested that
prominent HFSE depletion in similar basalts from the
Rio Grande rift is due to melting of asthenosphere
metasomatised by subduction-derived fluids above the
Farallon slab. Later Gibson et al. (1993)
argued that the source of these rocks was within the
lithosphere of a Proterozoic island-arc terrain. Recently,
the wide distribution of HAOTs in the western US were
interpreted with reference to a low-velocity zone (LVZ)
on top of the Farallon slab (Song et al.,
2004). This LVZ is probably a fluid-induced melting
zone (Song et al., 2004).
The Siberian Traps, which is the most
voluminous and probably the largest continental flood
basalt province (initiated in the latest Permian, but
volcanism continued up to the Middle Triassic; see Ivanov,
2007 for a review), was situated in the back-arc setting
of the Mongolia-Okhotsk and Paleo-Tethis oceans (see
Figure 5 in Ivanov, 2007 based on a
Late Perman paleotectonic reconstruction).
The southern (in present-day coordinates) part of the
Siberian Traps was about 700-800 km from the Mongolia-Okhotsk
trench. Trace-element compositions of the most Mg-rich
samples (the so-called low-Ti – 2 series) from
shallow sills practically overlap those of initial
melts of the Kamchatka arc (Figure 3), suggesting a
similar origin, i.e., melting of hydrated mantle).
Subduction signatures decrease with increasing distance
from the Mongolia-Okhotsk trench suggesting a link
with active (at time of magmatism) subduction.
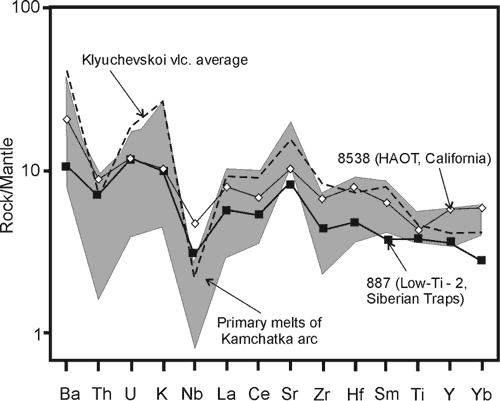
Figure 3. Comparison of the high-Mg-number
dolerite sample 887 of the low-Ti - 2 series with the
parental melt compositions of six different volcanoes,
including Klyuchevskoi volcano, along the frontal arc
of Kamchatka (100-180 km above the subducting slab)
(modified after Ivanov et al., in press). Other high
Mg-number samples of the Angara-Taseevskaya sills show
the same trace-element spectra and arenot plotted for
the sake of simplicity. Data for parental melts of
Kamchatka are plotted after Portnyagin et al. (2007).
The average of high Mg-number basalts of Klyuchevskoi
volcano (Dorendorf et al.,
2000) is also plotted to show that parental and crystallized
melts exhibit the same trace-element patterns. Rb,
Ta, Pb were not analyzed by Portnyagin et al. (2007)
and are thus excluded from the diagram. A HAOT sample
from California (after Grove et al., 2005)
is plotted to show that HAOT and low-Ti basalts are
geochemical analogues.
Conclusions
Western US, Karoo, the Siberian Traps
and other (e.g., Emeishan,
Columbia River Basalts) Late Paleozoic to Cenozoic
provinces with abundant low-Ti basalts should be revisited
to test whether their origins can be explained by associations
with subduction systems or if SCLM is indeed required
as commonly postulated. The true origin of volcanic
provinces with low-Ti basalts cannot be understood
until a multiple-working-hypothesis approach is used.
The postulate that low-Ti basalts are derived from
SCLM is based on the outdated assumption that subduction
geochemical features far from active subduction can
only be due to paleosubduction. Cold subduction can
bring significant amounts of water into the transition
zone. Consequent dehydration of the transition zone
is a possible explanation for subduction geochemical
signatures in volcanics beneath inner continental regions.
References
-
Arcay, D., Doin, M.-P., Tric, E., Bousquet, R., 2007.
Influence of postcollisional stage on subduction dynamics
and the buried crust thermal state: insights from numerical
simulations. Tectonophysics 441,
27-45.
-
Bina, C.R., Navrotsy, A., 2000. Possible presence
of high-pressure ice in cold subducting slabs. Nature 408,
844-847.
-
Bina, C.R., Stein, S., Marton, F.C., Van Ark,
E.M., 2001. Implications for slab mineralogy for
subduction dynamics. Phys. Earth Planet. Int. 127, 51-66.
-
Dorendorf,
F., Wiechert, U., Wörner, G., 2000.
Hydrated sub-arc mantle: a source for the Klyuchevskoy
volcano, Kamchatka/Russia. Earth Planet. Sci. Lett. 175, 69-86.
-
Gibson,
S.A., Thompson, R.N., Leat, P.T., Morrison, M.A.,
Hendry, G.L., Dickin, P., Mitchell, J.G.,
1993. Ultrapotassic magmas along the flanks of
the Oligo-Miocene Rio Grande rift, USA: monitors
of the zone of lithospheric mantle extension and
thinning beneath a continental rift. J. Petrol. 34,
187-228.
-
Grove, T.L., Baker, M.B.,
Price, R.C., Parman, S.W., Elkins-Tanton, L.T.,
Chatterjee, N., Müntener,
O., 2005. Magnesian andesite and dacite lavas from
Mt. Shasta , northern California: Products of fractional
crystallization of H2O rich mantle melts. Contrib.
Mineral. Petrol. 148, 542-565.
-
Hawkesworth,
C.J., Marsh, J.S., Duncan, A.R., Erlank, A.J., Norry,
M.J., 1984. The role of continental lithosphere in
the generation of the Karoo volcanic rocks: evidence
from combined Nd- and Sr-isotope studies. In: Petrogenesis
of the volcanic rocks of the Karoo province.(ed.) Erlank,
A.J. (Special Publication of the Geological Society
of South Africa 13), p. 341-354.
-
Hirschmann, M.M., Aubaud, C., Withers, A.C., 2005,
Storage capacity of H2O in nominally anhydrous minerals
in the upper mantle. Earth Planet. Sci. Lett. 236, 167-181
-
Ivanov,
A.V., 2007, Evaluation of different models for
the origin of the Siberian Traps. In: The origin
of melting anomalies: Plates, plumes and planetary
processes; (eds) Foulger G.R. and Jurdy
D.M., Geological Society of America Special Paper,
p. 669-692.
-
Ivanov,
A.V., Balyshev, S.V., 2005. Mass flux across the
upper-lower mantle boundary. In: Plates, plumes
and paradigms; (eds.) Foulger, G.R., Natland,
J.H., Presnall, D.C., Anderson, D.L., Geological
Society of America Special Paper 388, p. 327-346.
-
Ivanov,
A.V., Demonterova, E.I., Rasskazov, S.V., Yasnygina,
T.A., in press. Low-Ti melts from the Southeastern
Siberian Traps Large Igneous Province: Evidence for
a water-rich mantle source? J. Earth System Sci.
-
Komabayashi,
T., 2006. Phase relations of hydrous peridotite:
implications of water circulation in the Earth’s
mantle. In: Earth’s deep water cycle; (eds.)
Jacobsen, S.D., Van der Lee, S.,Geophysical monograph
168, p. 29-43.
-
Leat, P.T., Thompson, R.N., Morrison,
M.A., Hendry, M.A., Dickin, A.P., 1988. Compositionally
diverse Miocene-Recent rift-related magmatism in
Northwest Colorado: Partial melting and mixing of
mafic magmas from 3 different asthenospheric and
lithocpheric mantle sources. J.
Petrol. Special Lithosphere Issue, 351-377.
-
Litasov,
K, Ohtani, E., 2003. Stability of various hydrous
phases in CMAS pyrolite-H2O system up
to 25 GPa. Phys.
Chem. Minerals 30, 147-156.
-
Portnyagin,
M., Hoernle, K., Plechov, P., Mironov, N., Khubunaya,
S., 2007. Constraints on mantle melting and composition
and nature of slab components in volcanic arcs from
volatiles (H2O, S, Cl, F) and trace elements in melt
inclusions from the Kamchatka Arc. Earth
Planet. Sci. Lett. 255, 53-69.
-
Smyth, J.R., Frost, D.J., Nestola, F., Holl, C.M.,
Bromiley, G., 2006. Olivine hydration in the deep
upper mantle: Effect of temperature and silica activity. Geophys.
Res. Lett. 33, L15301.
-
Song,
T.-R.A., Helmberger, D.V., Grand, S.P., 2004. Low-velocity
zone atop the 410-km seismic discontinuity in the
northwestern United States. Nature 427, 530-533.
-
Ulmer,
P., 2001. Partial melting in the mantle wedge – the
role of H2O in the genesis of mantle-derived ‘arc-related’ magmas. Phys.
Earth. Planet. Interiors 127, 215-232.
-
Zhao, D., 2004. Global tomographic images of mantle
plumes and subducting slabs: insight into deep earth
dynamics. Physics Earth Planet. Int. 146,
3–34.
last updated 16th
December, 2007 |