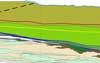 |
A 5-km-thick reservoir with >380,000 km3 of magma within the ancient Earth's crust
|
Rais Latypov1, Sofya Chistyakova1, Richard A. Hornsey2, Gelu Costin3, and Mauritz van der Merwe4
1School of Geosciences, University of the Witwatersrand, Johannesburg, South Africa; rais.latypov@wits.ac.za
2Richard Hornsey Consulting (Pty) Ltd, Paternoster, South Africa; richard.hornsey@gmail.com
3Department of Earth Science, Rice University, Houston, TX, USA; g.costin@rice.edu
4Retired, Port Alfred, South Africa; mauritzvdm1@gmail.com
This webpage is a summary of: Latypov, R., Chistyakova, S., Hornsey, R. A., Costin, G., and van der Merwe, M. (2022). A 5-km-thick reservoir with > 380,000 km3 of magma within the ancient Earth's crust, Scientific Reports 12, 1, 15651.
For over a century, the classic paradigm of volcanology and igneous petrology has been premised upon the existence of magma chambers, filled by crystal-free melt, forming ‘big tanks’ (Daly, 1911; Bowen, 1928; Wager & Brown, 1968; Parsons, 1987; Cawthorn, 1996; Marsh, 1996; Gudmundsson, 2012; Charlier et al., 2015). Such magma chambers gradually lose heat, crystallize from all margins inwards and occasionally supply overlying volcanoes with magma that erupts onto the Earth’s surface.
This founding concept has, however, been recently challenged on the basis of observations from various disciplines (Cashman et al., 2017; Bachmann & Huber, 2019; Sparks et al., 2019). The most often-cited evidence is from geophysical surveys that are unable to conclusively identify any present-day magma chambers with large volumes of eruptible melt (Lundstrom & Glazner, 2016). The conclusion from these studies is that large, predominantly molten magma chambers are likely either transient (Cashman et al., 2017) or non-existent (Coleman et al., 2004; Glazner et al., 2004) in the geological history of the Earth. As an alternative, it is proposed that intracrustal melt is stored within intergranular pockets of crystal-rich mushes that occupy almost the entire crust, from the Moho to the surface. Periodic tectonic destabilization of the mush is thought to produce small, discrete melt lenses that subsequently aggregate, ascend and erupt as lava (Cashman et al., 2017; Bachmann & Huber, 2019; Sparks et al., 2019).
Here we present field-based observations from the Bushveld Complex in South Africa that provide evidence to the contrary. A map of the south-eastern part of the complex (Figure 1) and an along-strike section through this area (Figure 2) reveal two notable features.
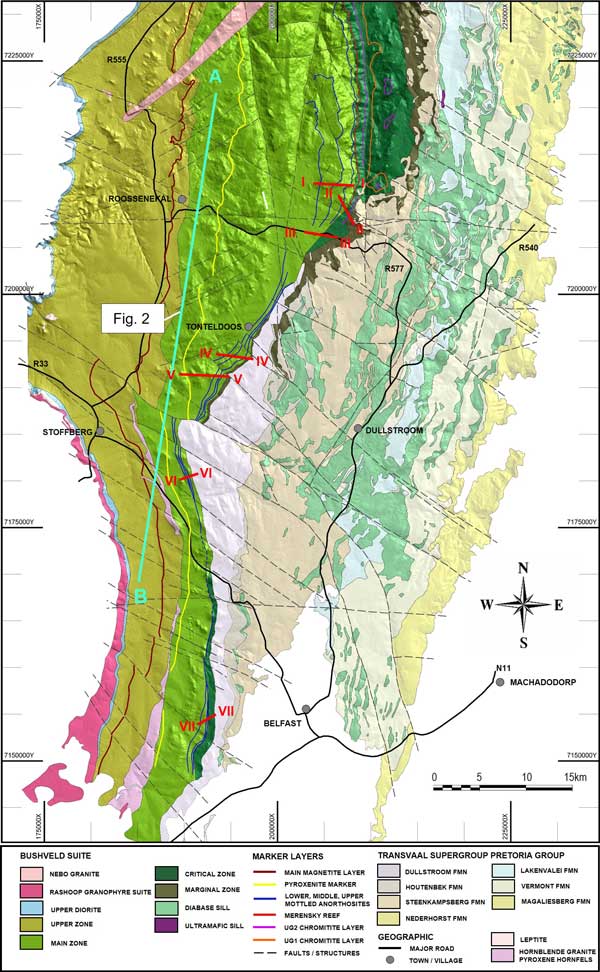
Figure 1: Geological map of the south-eastern part of the Bushveld Complex. The complex transgresses upwards through the Transvaal Supergroup over the northern 35 km of this sector and the floor of the complex steps up by approximately 6 km. The basal part of the Main Zone has three continuous markers layers termed the Lower, Middle, and Upper Mottled Anorthosites that extend along the entire area. The position of the cross-sections in Figure 2 and seven major traverses across the Anorthosite Markers are indicated.
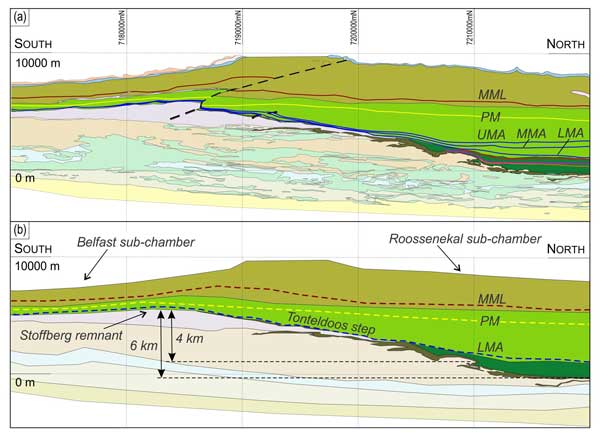
Figure 2: Geological along-strike section of the south-eastern part of the Bushveld Complex. (a) Section of the transect line AB in Figure 1, looking at -11° towards 270° azimuth. The section was constructed by rotating the 3D model to view the detailed geology of the complex and its immediate footwall in their true orientation. The section is not vertically or horizontally exaggerated and shows the true lithology morphology. (b) The schematic section prior to faulting that highlights the most important features of the complex. LMA, Lower Mottled Anorthosite; MMA, Middle Mottled Anorthosite; UMA, Upper Mottled Anorthosite; PM, Pyroxenite Marker; MML, Main Magnetite Layer. Other symbols as in Figure 1.
The first feature relates to the geometry of the chamber that is subdivided into the Roossenekal and Belfast sub-chambers (Figure 2). The floors to both sub-chambers are concave upwards and juxtapose an intervening Stoffberg remnant of non-deformed host rocks. The floor contact of the Roossenekal sub-chamber has an impressive ~6-km-high shelf relief (termed the Tonteldoos step; Figure 2b) with a ~10° slope across ~35 km up to the summit of the Stoffberg remnant.
The second feature relates to the igneous layering, defined by prominent layers termed the Lower, Middle and Upper Mottled Anorthosites (LMA, MMA and UMA). These occur close to the base of the Main Zone (MZ) of the complex. Importantly, the Anorthosite Markers drape the entire Roossenekal and Belfast sub-chambers, including the Stoffberg remnant. We have mapped the Anorthosite Markers along strike on seven field traverses through the basal part of the MZ in both sub-chambers (Figure 1). Importantly, the presence of the Anorthosite Markers across the entire area indicates that the deposition of MZ cumulates occurred synchronously across the deepest (i.e., Roossenekal sub-chamber base) and shallowest (i.e., Stoffberg remnant summit) parts of the sub-chamber (Figure 2). This occurred despite the elevation difference between the two depositional sites being ~4.0 km (Figure 2b).
Igneous layering in mafic intrusions results from deposition of crystals from the overlying resident melt by gravity settling (Wager & Brown, 1968; Irvine, 1980; Morse, 1986) or in situ crystallization (Campbell, 1978, 1996; McBirney & Noyes, 1979; Latypov et al., 2015, 2020) onto the chamber floor. In order to blanket the topographic relief of a temporary floor of the Bushveld chamber with igneous layering (i.e., LMA), the resident melt column must have been thicker than the ~4.0 km height of the Tonteldoos step. Prolonged internal differentiation within a thick magma column in this area is further supported by evolutionary trends in crystallization sequence and mineral compositions through the sequence (Von Gruenewaldt, 1973).
Based on both field and chemical observations, the total thickness of the melt column of the MZ is estimated at ~5.0 km. Rather than implying that the entire ~5.0 km thick column formed from a single large magma influx, it is proposed that the intrusion progressively grew to its final size through emplacement of numerous magma influxes, yet over a much shorter time scale than solidification. During this period of repeated injections, each replenishment effectively mixed with the resident melt in the chamber, thereby delaying or impeding the onset of crystallization. Thus, crystallization commenced within a completely filled, large and homogenized magma chamber, which can thereby be modelled as having crystallized as a ‘single pulse’ of magma (Figure 3).
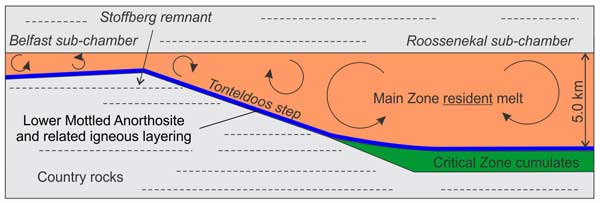
Figure 3: An emplacement model for the Main Zone in the south-eastern part of the Bushveld Complex. The Main Zone progressively grew to its final size through emplacement of numerous magma influxes which effectively mixed with the resident melt in the chamber, thereby delaying or impeding the onset of crystallization. Thus, crystallization finally commenced within a large and homogenized magma chamber, with a melt column having a total thickness of about 5 km. This resulted in simultaneous deposition of the Lower Mottled Anorthosite (LMA) along the entire extent of the Roossenekal and Belfast sub-chambers, including the Tonteldoos step and Stoffberg remnant. The total volume of resident melt before onset of crystallization is estimated at >380,000 km3 which allows classification of the Bushveld chamber as a ‘big tank’ open-system within the Earth’s crust.
The 5-km-thick resident melt column that produced the chemically stratified MZ cumulate sequence, including its three prominent Anorthosite Markers, thus indicates that during this stage the Bushveld chamber was exceptionally large and entirely molten (Figure 3). This conclusion is at odds with the emerging paradigm that such ‘big tank’ magma chambers were ephemeral at any given time throughout Earth’s geological history (Coleman et al., 2004; Glazner et al., 2004; Cashman et al., 2017).
The total volume of resident MZ melt may be estimated as follows. The MZ melt column thickness varied between ~5.0 km in the thicker parts of the intrusion and at least 1.0 km in the thinner parts. Based on reconstructions of the lateral extent of the MZ (Kruger, 2005), it is estimated that the thicker areas occupied ~70% of the MZ, and the remaining ~30% were thinner zones. The estimated Bushveld Complex area is approximately 100,000 km3 (Cawthorn & Walraven, 1998) and therefore the total volume of the MZ resident melt is ~380,000 km3 (5 km * 70,000 km2 + 1.0 km * 30,000 km2).
This volume is several orders of magnitude larger than the largest ignimbrite/tuff super-eruptions in Earth’s history (e.g., the Bishop tuff – 600 km3 and the Youngest Toba eruption – up to 13,200 km3) (Miller & Wark, 2008). It is only comparable to estimates of some of Earth’s large igneous provinces, such as the Karoo (367,000 km3) (Svensen et al., 2014) and Afar (350,000 km3) (Ross et al., 2005). Thus, during emplacement of the MZ, the Bushveld magma chamber was a repository of an enormous volume of resident melt and may be regarded as a ‘big tank’ open-system within the Earth’s crust.
The current tendency in modern volcanology (Coleman et al., 2004; Glazner et al., 2004; Cashman et al., 2017; Bachmann & Huber, 2019; Sparks et al., 2019) to reject the possible existence of such large and molten magma chambers (Wager & Brown, 1968; Parsons, 1987; Cawthorn, 1996; Charlier et al., 2015) therefore appears to be premature. It is conceivable that such magma chambers developed throughout the entire Earth’s evolution. Moreover, since layered intrusions such as the Bushveld Complex are rare throughout geological time (Smith & Maier, 2021), it is not surprising that there are currently no active examples of large and molten magma chambers in Earth’s crust which have been detected geophysically (Lundstrom & Glazner, 2016).
References
- Bachmann, O. & Huber, C. (2019). The inner workings of crustal distillation columns; the physical mechanisms and rates controlling phase separation in silicic magma reservoirs. Journal of Petrology 60, 3–18.
- Campbell, I. H. (1978). Some problems with the cumulus theory. Lithos 11, 311–323.
- Campbell, I. H. (1996). Fluid dynamic processes in basaltic magma chambers. Developments in Petrology. Elsevier, 45–76.
- Cashman, K. V., Sparks, R. S. J. & Blundy, J. D. (2017). Vertically extensive and unstable magmatic systems: a unified view of igneous processes. Science 355, eaag3055.
- Cawthorn, R. G. (1996). Layered Intrusions. Elsevier, Amsterdam.
- Cawthorn, R. G. & Walraven, F. (1998). Emplacement and crystallization time for the Bushveld Complex. Journal of Petrology 39, 1669–1687.
- Charlier, B., Namur, O., Latypov, R. & Tegner, C. (2015). Layered Intrusions. Dordrecht: Springer Netherlands.
- Coleman, D. S., Gray, W. & Glazner, A. F. (2004). Rethinking the emplacement and evolution of zoned plutons: geochronologic evidence for incremental assembly of the Tuolumne Intrusive Suite, California. Geology 32, 433.
- Glazner, A. F., Coleman, D. S., Gray, W. & Taylor, R. Z. (2004). Are plutons assembled over millions of years by amalgamation from small magma chambers? GSA TODAY 14, 4–12.
- Irvine, T. N. (1980). Magmatic density currents and cumulus processes. American Journal of Science 280-A, 1–58.
- Kruger, F. J. (2005). Filling the Bushveld Complex magma chamber: lateral expansion, roof and floor interaction, magmatic unconformities, and the formation of giant chromitite, PGE and Ti-V-magnetitite deposits. Mineralium Deposita 40, 451–472.
- Latypov, R., Chistyakova, S., Page, A. & Hornsey, R. (2015). Field evidence for the in situ crystallization of the Merensky Reef. Journal of Petrology 56, 2341–2372.
- Latypov, R. M., Chistyakova, S. Yu., Namur, O. & Barnes, S. (2020). Dynamics of evolving magma chambers: textural and chemical evolution of cumulates at the arrival of new liquidus phases. Earth-Science Reviews 210, 103388.
- Lundstrom, C. C. & Glazner, A. F. (2016). Silicic magmatism and the volcanic–plutonic connection. Elements 12, 91–96.
- McBirney, A. R. & Noyes, R. M. (1979). Crystallization and layering of the Skaergaard intrusion. Journal of Petrology 20, 487–554.
- Miller, C. F. & Wark, D. A. (2008). Supervolcanoes and their explosive supereruptions. Elements 4, 11–15.
- Morse, S. A. (1986). Convection in aid of adcumulus growth. Journal of Petrology 27, 1183–1214.
- Parsons, I. (1987). Origins of Igneous Layering. Springer, Dordrecht.
- Ross, P.-S., Ukstins Peate, I., McClintock, M. K., Xu, Y. G., Skilling, I. P., White, J. D. L. & Houghton, B. F. (2005). Mafic volcaniclastic deposits in flood basalt provinces: A review. Journal of Volcanology and Geothermal Research 145, 281–314.
- Smith, W. D. & Maier, W. D. (2021). The geotectonic setting, age and mineral deposit inventory of global layered intrusions. Earth-Science Reviews 220, 103736.
- Sparks, R. S. J., Annen, C., Blundy, J. D., Cashman, K. V., Rust, A. C. & Jackson, M. D. (2019). Formation and dynamics of magma reservoirs. Philosophical Transactions of the Royal Society A: Mathematical, Physical and Engineering Sciences 377, 20180019.
- Svensen, H. H., Polteau, S., Cawthorn, G. & Planke, S. (2014). Sub-volcanic Intrusions in the Karoo Basin, South Africa. In: Breitkreuz, C. & Rocchi, S. (eds) Physical Geology of Shallow Magmatic Systems. Cham: Springer International Publishing, 349–362.
- Von Gruenewaldt, G. (1973). The Main and Upper zones of the Bushveld Complex in the Roossenekal area, eastern Transvaal. Geological Society of South Africa Transactions 76, 53–61.
- Wager, L. R. & Brown, G. M. (1968). Layered igneous rocks. Edinburgh: Oliver & Boyd, Edinburgh & London
last updated 25th September, 2022 |