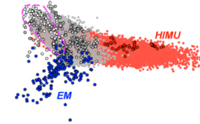 |
Monte-Carlo simulations of metasomatic enrichment in the lithosphere and implications for the source of alkaline basalts |
Sébastien Pilet
Institute of Earth Sciences, University of Lausanne, 1015 Lausanne, Switzerland; Sebastien.Pilet@unil.ch
This webpage is a summary of: Pilet S., Baker M.B., Müntener O. and Stolper, E.M. Monte-Carlo simulations of metasomatic enrichment in the lithosphere and implications for the source of alkaline basalts, Journal of Petrology 52, 1415-1442, 2011
Introduction
Nepheline (ne)-normative alkaline lavas from oceanic and continental intraplate volcanoes are characterized by fractionated incompatible trace-element contents higher than those found in MORBs (Gast, 1968). This high trace-element contents has been explained by low degrees of partial melting of a garnet peridotite source, but the TiO2 contents of OIBs suggest that the sources of these lavas have TiO2 concentrations higher than those estimated for primitive mantle (Prytulak & Elliott, 2007), which in turn suggests that other incompatible minor and trace elements may also be enriched in these sources. Such inferences are consistent with the need for trace-element enriched components in the sources of OIBs to explain the range of their radiogenic isotopic compositions (Gast et al., 1964; Chase, 1981; Hofmann & White, 1982; Zindler & Hart, 1986). One hypothesis to explain the formation of trace-element enriched alkaline lavas is that these lavas are produced by low degree melts from an asthenospheric source containing recycled oceanic crust ± sediments (Barling & Goldstein, 1990; Chase, 1981; Chauvel et al., 1992; Hart, 1988; Hofmann & White, 1982; Nakamura & Tatsumoto, 1988; Palacz & Saunders, 1986; Weaver, 1991). An alternative is that they represent the melts of amphibole-rich metasomatic veins in the lithosphere or melts of their dehydrated equivalents if metasomatized lithosphere is recycled into the convecting mantle (Halliday et al., 1992; Halliday et al., 1995; Niu & O'Hara, 2003; Panter et al., 2006; Pilet et al., 2008; Pilet et al., 2005; Sun & McDonough, 1989; Workman et al., 2004).
The metasomatic hypothesis for the origin of alkaline lavas
Melting experiments on amphibole-bearing veins indicate that high-degree melting of metasomatic veins followed by variable amounts of interaction with surrounding mantle can reproduce key features of the major- and trace-element compositions of alkaline magmas (Figure 1, modified from Figures 1 and 3 of Pilet et al., 2008). Two scenarios have been proposed for the production of alkaline magmas by the melting of metasomatized lithosphere:
- Shortly after, or coincident with, metasomatism the lithosphere experiences a thermal perturbation or decompression and melts in situ (Halliday et al., 1990; Lloyd & Bailey, 1975; Pilet et al., 2008; Wass & Rogers, 1980); or
- Metasomatized lithosphere is recycled into the convecting mantle by subduction or delamination and melts during later upwelling (e.g., in a plume; Halliday et al., 1995; McKenzie & O'Nions, 1995; Niu & O'Hara, 2003; Pilet et al., 2004, 2008).
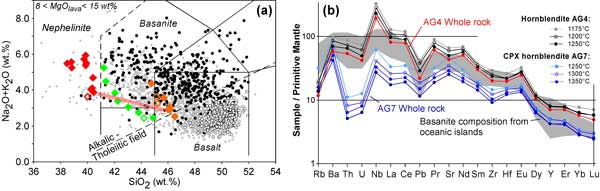
Figure 1: Main results of the experimental study on partial melting of amphibole-bearing veins at 1.5 GPa (Pilet et al., 2008). (a) Total alkali vs. SiO2 (volatile-free) for hornblendite, clinopyroxene-hornblendite, and sandwich experiments (hornblendite + peridotite) compared to continental and oceanic intraplate basalts and mid-ocean-ridge basalt (MORB) compositions. Solid gray circles, OIBs; solid black circles, continental intraplate basalts; open black circles, MORBs. Solid red and green diamonds, glass compositions from 1.5-GPa experiments on AG4 and AG7, respectively (the open diamonds are the starting compositions). Solid and open orange diamonds, glass compositions from 1.5 GPa sandwich experiments where orthopyroxene is present or absent, respectively, in the peridotitic layers. The orange line connects the AG4 melt compositions to the compositions of glasses produced at the same temperature (1300°C) in the sandwich experiments (AG4 + peridotite) and illustrates how melt compositions change with the assimilation of orthopyroxene (+ spinel) from the peridotite layers. (b) Primitive mantle-normalized trace-element abundances for hornblendite AG4 and clinopyroxene hornblendite (AG7) melts. The gray band shows the range (defined as ± 1σ of the average of 195 analyses) of trace-element contents in low-silica OIB lavas (40 to 44 wt% SiO2) with 8 to 15 wt% MgO (compiled from the GEOROC database). Click here or on Figure for enlargement.
Both scenarios suggest that alkaline magmas are produced by high degrees of melting (>40%) of hydrous metasomatic veins or their dehydrated equivalents (Pilet et al., 2008), and thus the incompatible trace-element contents of the alkaline melts primarily reflect the compositional characteristics of the metasomatic source material. This is an important aspect of the metasomatic model. Some features considered diagnostic of intraplate alkaline magmas are simply inherited from the metasomatic source lithologies. Consequently, testing of the metasomatic model requires evaluating whether these characteristics are indeed expected.
To evaluate whether such metasomatic veins are a natural consequence of metasomatism within the lithosphere, we constructed a forward model of vein formation and subsequent melting in the lithosphere. Although such petrogenetic modeling is typically done using inverse techniques, the sequential nature of the processes involved in metasomatic vein formation dictate a forward approach. Since our forward model involves a large number of adjustable parameters with wide ranges of acceptable values (e.g., the origin of the metasomatic fluid/melt, identity and proportions of crystallizing phases, trace-element partition coefficients, time-delay between metasomatism and melting, etc.), we used Monte Carlo methods to investigate how the uncertainties on these parameters affect the degree to which melting of metasomatized lithospheric sources are consistent with the trace-element contents and isotopic ratios of intraplate alkaline magmas.
A model for the formation of metasomatic veins
Figure 2 shows schematically the model we adopt in this study. The starting point is the generation of liquids by low degrees of partial melting at high pressures below a parcel of lithosphere. This could occur in a variety of environments (Figure 2) including small degrees of “wet” melting during normal upwelling beneath a mid-ocean ridge (Class & Goldstein, 1997; Halliday et al., 1995; Niu & O'Hara, 2003) at the periphery of an ascending plume beneath continental or oceanic lithosphere (Halliday et al., 1995; Wyllie, 1988a, 1988b) or during upwelling of the asthenosphere beneath rifting lithosphere (Lloyd & Bailey, 1975; Pilet et al., 2004; Thompson et al., 2005; Wass & Rogers, 1980). These low-degree melts then ascend into the lithosphere, metasomatizing it as they interact chemically with their surroundings. They form veins by precipitation of the phases that crystallize from them progressively as they cool (Harte et al., 1993; McKenzie, 1989; Morris & Pasteris, 1987; Nielson & Noller, 1987; Wilshire, 1987). Alkaline melts are then formed by melting of the assemblage of veins and metasomatized peridotite.
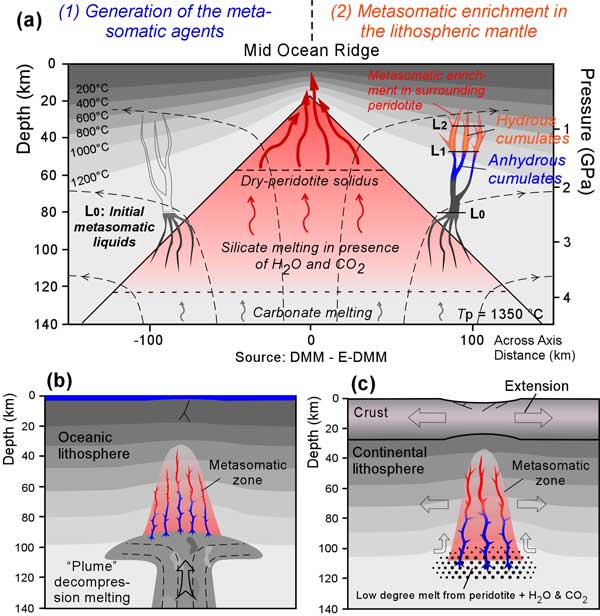
Figure 2: (a) Schematic model for lithospheric metasomatism at the periphery of a mid-ocean ridge. This is based on models suggested by Halliday et al. (1995) and Niu & O’Hara (2003). It assumes that near-solidus liquids produced at depth in the melting column are not collected to form MORB, but percolate across the cooling lithosphere and generate anhydrous and hydrous cumulate veins plus cryptic and modal metasomatic enrichment in the adjacent peridotite. The geometry and thermal structure of the cooling lithosphere is based on the model published by Shaw et al. (2010) for the Gakkel Ridge. (b) Schematic model for the metasomatism of oceanic lithosphere induced by a mantle plume. Some melts produced by plume upwelling do not reach the surface but percolate through and differentiate within the oceanic lithosphere producing anhydrous and hydrous cumulates plus metasomatic enrichment in the lithospheric peridotite surrounding these cumulates (Wyllie,1988a, 1988b; Halliday et al.,1995). (c) Schematic model for the metasomatism of the continental lithosphere. The generation of asthenospheric melts responsible for the formation of metasomatic veins and cryptic and modal metasomatism in adjacent peridotite surrounding the veins is due to upwelling of the asthenosphere beneath rifting lithosphere (Lloyd & Bailey, 1975; Wass & Rogers, 1980; Pilet et al., 2004; Thompson et al., 2005) or to ‘finger-like’ plumes (Granet et al., 1995; Hoernle et al., 1995; Wilson & Patterson, 2001). (See Figure 3 for a discussion of L0, L1, and L2, and for the colour-scale coding of the schematic veins.)
Figure 3 illustrates the two steps of the model:
-
Formation of a low-degree partial melt of garnet peridotite in the presence of volatiles, and
-
Differentiation of this liquid as it moves through the lithosphere, generating different metasomatic veins as it ascends and cools.
Metasomatic veins are interpreted as cumulates formed during the fractional crystallization of volatile-rich melts within the lithosphere (Bodinier et al., 1987, 2004; Downes, 2007; Harte et al., 1993; Morris & Pasteris, 1987; Nielson & Noller, 1987; Nielson & Wilshire, 1993; Wilshire, 1987). Although the compositions of the melts that produce metasomatic veins in the lithosphere are poorly constrained, they are generally thought to be low-degree partial melts of H2O+CO2-bearing mantle peridotite. Migration, reaction, cooling, and fractional crystallization of these low-degree melts in the lithospheric mantle generates a continuum of phase assemblages ranging from anhydrous (clinopyroxene (cpx) + garnet (gt) ± olivine (ol) ± orthopyroxene (opx)) to hydrous (cpx + amphibole (amph) ± phlogopite (phlog) ± opx) veins enriched in highly incompatible elements, plus a modal and cryptic enrichment in the surrounding peridotite (Harte et al., 1993; McPherson et al., 1996; Morris & Pasteris, 1987; Nielson & Noller, 1987; Nielson & Wilshire, 1993; Wilshire, 1987). An important point is that the initial liquid which produces the different cumulate is insensitive to whether the melt is ne- or hy-normative (Pilet et al., 2010). Amphibole cumulates similar in composition to those in metasomatic veins can be produced by differentiation of a liquid similar to the partial melt of peridotite with a volatile content characteristic of the upper mantle, regardless of whether the liquid is ne- or hy- normative.
A summary of the parameters describing these processes is given in Figure 3. See Pilet et al. (2011) for a complete description of the parameters and Kds we used. We have estimated both the parameters and their uncertainties using experimental, petrologic, and geochemical constraints and have used Monte-Carlo simulations to understand how these uncertainties propagate through the calculation and impact the calculated compositions of potential metasomatic sources.
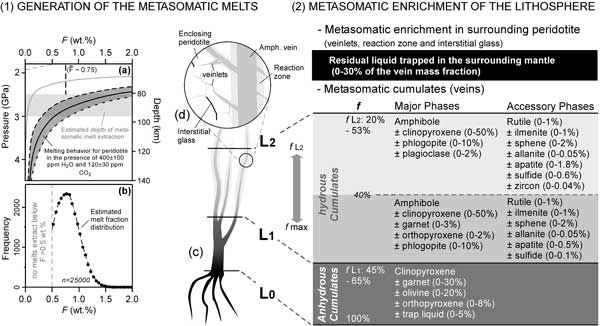
Figure 3. Parameters used to calculate (1) the initial melt composition that will metasomatize the lithosphere, and (2) the composition of metasomatized lithosphere. (a) Relationship between depth and melt fraction (F) for isentropic melting of peridotites containing various amounts of H2O and CO2. These melting curves are calculated based on the parameterization of Dasgupta et al. (2007) for the combined effect of H2O and CO2 on peridotite melting temperatures and using a potential adiabat temperature of 1350°C. The gray band shows our estimate for the depths of metasomatic melt extraction. (b) Frequency distribution of melt fraction for asthenospheric melting used in our Monte Carlo simulations. (c) Phase proportions used in the Monte Carlo simulations. The percentages listed after each mineral show the bounds within which the proportions in the cumulate assemblage were free to vary. f is the proportion (in %) of residual liquid. The amount of residual liquid at the transition between anhydrous and hydrous cumulate formation (f L1) is free to vary between 65 and 45%, whereas the amount of residual liquid (f L2) at the end point of the calculation can vary from 53% to 20%. The compositions of hydrous cumulates are randomly calculated between fmax and f L2. The proportion of clinopyroxene in the anhydrous cumulates and amphibole in the hydrous cumulates was calculated by difference. The proportion of phlogopite (0-10%) in the veins represents the potential presence of veinlets in association with major metasomatic veins [see (d)]. The proportion of trapped liquid in the surrounding peridotite is linked to the proportion of phlogopite in the veins assuming that veinlets and metasomatism in the surrounding peridotite correspond to a unique process (the ratio of phlogopite to trapped liquid is 1:3). (d) Schematic illustration of progressive infiltration of metasomatic melts within surrounding peridotite [modified from Figure 1 of Wulff-Pedersen et al. (1999)]. (N.B. The model developed here for alkaline magma generation in continental settings is valid only for regions where the lithosphere is ~100 km thick. For thicker lithosphere the sequence and mode of fractionating phases is likely to be different from that used here.) Click here or on Figure for enlargement.
Comparison of natural alkaline magmas and model of metasomatized hydrous lithosphere
The results of our modelling are shown in Figure 4. The calculations show that the trace-element patterns of the near-solidus peridotite melts (L0, Figure 4a), the model coexisting liquid (L1 to L2; Figure 4b), and the initial model anhydrous cumulate assemblage (clinopyroxene ± garnet ± olivine ± orthopyroxene; Figure 4c) do not match the patterns observed in alkaline lavas. Conversely, further crystallization and the appearance of amphibole (and accessory minerals such as rutile, ilmenite, apatite, etc.) produce model hydrous cumulate assemblages (including 0 to 30% of trapped liquid mimicking the cryptic and modal metasomatism surrounding metasomatic veins) characterized by trace-element patterns that closely match those observed in alkaline lavas. These calculated hydrous cumulate assemblages are highly enriched in incompatible trace elements and share many similarities with the trace-element patterns of alkaline basalt observed in oceanic or continental setting (positive Nb/La, negative Ce/Pb, similiar slopes of REEs). The high concentration of incompatible elements in the hydrous cumulates reflects:
-
The relative high Damph/liqs for most of these elements (exceptions are U, Th and Pb), and
- Accessory minerals in the hydrous cumulates such as apatite, allanite, sphene, and zircon (where many of these trace elements reach very high concentrations).
Figure 4: (a) Trace-element contents (normalized to primitive mantle, PM; McDonough & Sun, 1995) of model-generated liquids produced by low-degree melting of the asthenosphere compared with continental and oceanic basanites and the assumed asthenospheric mantle end-members (green band: DMM to E-DMM; Workman & Hart, 2005). L0 is the composition of unfractionated H2O and CO2-bearing near-solidus peridotite melt. This composition corresponds to the initial melt entering the lithosphere from the underlying asthenosphere. The liquid labeled F = 0·2 wt % represents a lower partial melting calculation. It is also shown in order to demonstrate that even at a lower degree of partial melting a DMM source cannot produce the composition of alkaline lavas observed in oceanic islands. (b) Model-generated trace-element contents (normalized to PM) of the average L0 [black curve from (a)] of residual liquids L1 (blue) and L2 (red) after fractionation of anhydrous and anhydrous + hydrous cumulates respectively (see also Figure 3). (c) Model-generated trace-element contents (normalized to PM) of calculated anhydrous (blue) and metasomatized hydrous lithosphere including various proportions of trapped liquid (0-30%) in the peridotite surrounding the vein (red). The gray bands in (a) - (c) show the compositions of basanites from oceanic and continental intraplate volcanoes compiled from the GeoRoc database. For this compilation only basalts characterized by ne-normative compositions with SiO2 contents between 40 and 45 wt % and with MgO contents between 8 and 15 wt % were selected. (d) Trace-element contents (normalized to PM) of amphiboles from the model hydrous cumulates (dark red curve) compared with amphibole compositions from metasomatic veins observed in oceanic and continental settings (Gray lines; Ionov & Hofmann, 1995; Vaselli et al., 1995; Zanetti et al., 1996; Wulff-Pedersen et al., 1999; Moine et al., 2001; Downes et al., 2004; Pilet et al., 2008). For the model calculations, the dark-colored curves in each panel correspond to the average compositions of the liquids or cumulates, whereas the surrounding lighter-colored bands denote the ±1σ range for the corresponding colored curves. The long and short dashed lines in each panel represent the maximum and minimum compositions, respectively, calculated in the simulations. Click here or on Figure for enlargement.
The presence of trapped liquid surrounding the veins has a limited effect on the global trace-element enrichment of hydrous metasomatic lithosphere, but could modify the relative abundance of Rb, Ba, U, Nb and Pb regarding other incompatible elements such Nb or LREE. A comparison of melts from hydrous metasomatic vein compositions (calculated at F = 50%) with alkaline OIBs (Figure 5) indicates that it is possible to reproduce the variations in Ba/Nb, Ce/Pb and Nb/U observed in alkaline basalt worldwide by varying the proportion of trapped liquid in the hydrous metasomatic lithospheric source.
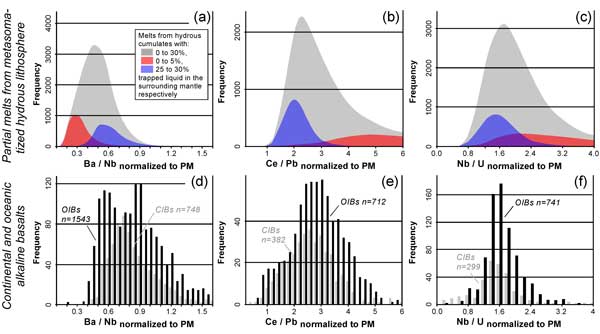
Figure 5. (a-c) Distributions of trace-element ratios (a, Ba/Nb; b, Ce/Pb; c, Nb/U; all normalized to PM, (McDonough & Sun, 1995) in 50% partial melt of metasomatized hydrous lithosphere containing 0-5% (red area), 0-30% (gray area), and 25-30% (blue area) trapped liquid in the surrounding peridotite. (d-f) Distributions of PM-normalized trace-element ratios (d, Ba/Nb; e, Ce/Pb; f, Nb/U) in alkaline OIB (black lines) and alkaline continental intraplate basalts (CIB; gray lines) selected from the GeoRoc database. All basalts shown in (d) - (f) have normative nepheline, MgO contents between 6 and 20 wt%, and for continental lavas, K2O/Na2O weight ratios <1. Click here or on Figure for enlargement.
One critical aspect of our calculation is that amphibole compositions predicted by the model are in agreement with the compositions of amphibole observed in metasomatic veins worldwide (Figure 4d). This suggests that the model is realistic. We conclude from these calculations that amphibole-bearing veins represent a potential source in terms of major- (see Pilet et al., 2008) and trace elements to produce, at moderate to high degrees of partial melting, the composition of alkaline rocks observed in intraplate volcanoes.
Isotopic evolution of metasomatized hydrous lithosphere over time
As mentioned above, two scenarios have been proposed for the production of alkaline magmas by melting of metasomatized lithosphere (Halliday et al., 1995; Niu & O’Hara, 2003; Pilet et al., 2004, 2005, 2008):
- Shortly after or coincident with metasomatism, the lithosphere experiences a thermal perturbation or decompression and thereby melts in situ; or
- The metasomatized lithosphere is recycled into the convecting mantle by subduction or delamination and melts during later upwelling (e.g. in a plume or at a ridge).
As for Ba/Nb, Ce/Pb and Nb/U (Figure 5), models for metasomatized hydrous lithosphere predict highly variable parent-daughter ratios for elements that control the evolution of the major radiogenic isotope systems (e.g., Rb/Sr, Sm/ Nd, and U/Pb). These predictions could be used to evaluate the temporal evolution of radiogenic isotope ratios depending on the mineralogy of the veins, the amount of trapped liquid in the surrounding peridotite, and the time interval between vein formation and melting.
The range of isotopic compositions calculated for a metasomatized lithosphere isolated for 0.15 Gyr (gray dots in Figure 6), time corresponding to potential residence time of oceanic lithosphere at Earth surface before subduction is significantly larger than the compositions exhibited by MORB (which is taken as a proxy for the isotopic composition of the ‘zero-age’ veins–purple lines in Figure 6) and partially overlaps the compositional range observed in alkaline OIBs. Many alkaline OIBs have isotopic compositions that lie within the following ranges: 18 <206Pb/204Pb< 20, 0·702 <87Sr/86Sr< 0·704, and 0·5127 <143Nd/144Nd< 0·5132 (as opposed to the more extreme values shown by EM or HIMU basalts), and it is the OIBs with these "normal" isotopic compositions that are consistent with our model of a metasomatized lithospheric source that has evolved for 0.15 Gyr. Significantly, only OIBs characterized by EM or HIMU isotopic signatures cannot be explained by the melting of metasomatized lithosphere in situ, assuming that the composition of the source producing the veins is similar to DMM to E-DMM.
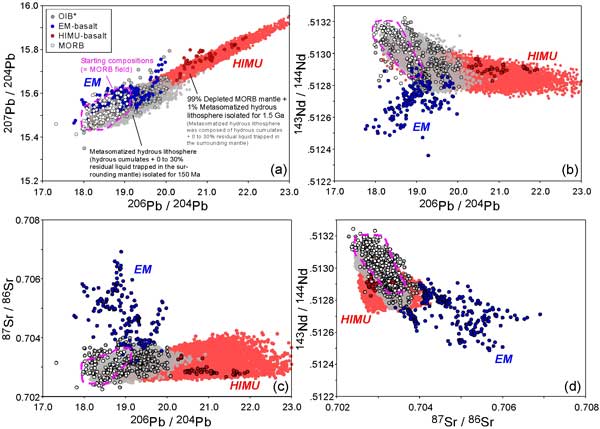
Figure 6. Isotopic compositions of OIB compared with the calculated isotope ratios of our Monte Carlo model-generated metasomatized hydrous lithosphere (hydrous cumulates plus 0-30% trapped liquid in the surrounding peridotite) isolated for 150 Ma (gray dots), and for metasomatized peridotite (1% metasomatized hydrous lithosphere + 99% depleted mantle) isolated for 1.5 Ga (red dots). OIB data were selected from the GeoRoc database. All rocks are ne-normative and have MgO contents between 6 and 20 wt%. HIMU (red dots) and EM (blue dots) basalts are distinguished from "normal’"OIB (OIB*; gray dots) based on their isotopic compositions (EM if 143Nd/144Nd <0·51275 or 87Sr/86Sr >0·704; HIMU if 206Pb/204Pb >20·3). MORB data (white circles) are taken from the PetDB database. Click here or on Figure for enlargement.
Given that the isotopic ratios of the HIMU and EM end-members shown in Figure 6a to 6d cannot be explained by short times operating on enriched metasomatic veins, we now ask whether longer times might be able to explain these isotopic end-members in the context of our model. Chase (1981), Weaver (1991), and Chauvel et al. (1992) have previously suggested that the sources of EM and HIMU lavas have been isolated for long periods of time. If metasomatized lithosphere is recycled through the convecting mantle and entrained in mantle plumes or larger-scale upwellings (e.g., beneath oceanic ridges), then the metasomatic veins characterized by high Rb/Sr, Sm/Nd, and U/Pb would evolve to extreme Sr, Nd, and Pb isotopic signatures if the veins were in fact isolated for time scales of the order of 1-2 Gyr. A significant issue for this type of calculation is, however, whether the heterogeneities associated with metasomatized lithosphere (e.g., veins in mantle outcrops in the French Pyrenees are of the order of 0.1 - 1 m in width) could survive mechanical stirring and diffusive homogenization in the convecting mantle on such time scales (Kogiso et al., 2004). For this reason, we calculated the isotopic evolution of a source that represents a mixture of 99% depleted mantle (DMM) plus 1% metasomatized hydrous lithosphere (veins plus various proportions–0-30%–of trapped liquid). Figure 6 shows how the radiogenic isotopic composition of this mixture evolves over 1.5 Gyr (red dots). Our calculations indicate that a large range of isotopic compositions can be produced by isolating the metasomatized lithospheric mantle for ~1-2 Gyr. These calculations support the idea that recycling in subduction zones or delamination of oceanic and/or continental metasomatized lithosphere could yield enriched mantle with isotopic compositions similar to those inferred for the HIMU end-member. Only basalt characterized by an EM isotopic composition would be difficult to reproduce by long-term isolation of a metasomatic mantle component. The U/Pb ratio calculated for metasomatized lithosphere is too high to produce the Pb isotopic compositions observed in lavas characterized by an EM signature.
Concluding remarks
Our Monte Carlo simulations of metasomatic enrichment within the lithosphere show the following results.
- The high- and fractionated trace-element contents of hydrous metasomatic veins are consistent with these veins representing cumulates produced by fractional crystallization within the lithosphere of low-degree melts from an asthenospheric source similar to that of MORB (i.e. the veins do not represent liquid compositions).
- Details of the trace-element patterns observed in these veins (e.g., the positive Nb/La and Ce/Pb ratios; Figure 4c) are not inherited from an initial low-degree melt of the asthenosphere but instead largely reflect partitioning between amphibole and melt during fractionation of mantle-derived melts as they traverse the lithosphere and as amphiboles accumulate in the conduits to form veins.
- Partial melts of amphibole-rich cumulates based on our models (particularly when the effects of cryptic and modal metasomatism of the peridotitic country rock are included and contribute to the melts) provide good matches to the trace- and minor-element abundances and patterns of basic alkaline magmas (e.g., basanites) from oceanic and continental settings.
- Sr, Nd, and Pb radiogenic isotope ratios from time-evolved amphibole-rich cumulates predicted by the Monte Carlo models (+ metasomatized adjacent peridotite + primitive peridotite) are consistent with the range of values observed in most alkaline OIB and continental magmas. HIMU magmas require ca. 1-2 Gyr of evolution. EM magmas cannot be readily explained without appealing to other factors such as a heterogeneous asthenosphere containing recycled sediments.
Based on these results, the differentiation of low-degree melts from a normal “MORB” peridotitic source across the lithospheric mantle can produce metasomatic amphibole-bearing cumulates suitable as a source for alkaline magmas in term of major- and trace elements. Melting of metasomatized veined lithosphere in situ or after recycling through the convecting mantle is a viable hypothesis for the formation of the alkaline basalts observed in continental or oceanic settings.
References
-
Barling, J. & Goldstein, S. L. (1990). Extreme isotopic variation in Heard-island lavas and the nature of mantle reservoirs. Nature 348, 59-62.
-
Bodinier, J. L., Fabries, J., Lorand, J. P., Dostal, J. & Dupuy, C. (1987). Geochemistry of amphibole pyroxenite veins from the Lherz and Freychinede ultramafic bodies (Ariege, French Pyrenees). Bulletin de Mineralogie 110, 345-358.
-
Bodinier, J. L., Menzies, M. A., Shimizu, N., Frey, F. A. & McPherson, E. (2004). Silicate, hydrous and carbonate metasomatism at Lherz, France: Contemporaneous derivatives of silicate melt-harzburgite reaction. Journal of Petrology 45, 299-320.
-
Chase, C. G. (1981). Oceanic Island Pb—2-stage histories and mantle evolution. Earth and Planetary Science Letters 52, 277-284.
-
Chauvel, C., Hofmann, A. W. & Vidal, P. (1992). HIMU and EM—the French-Polynesian connection. Earth and Planetary Science Letters 110, 99-119.
-
Class, C. & Goldstein, S. L. (1997). Plume-lithosphere interactions in the ocean basins: constraints from the source mineralogy. Earth and Planetary Science Letters 150, 245-260.
-
Dasgupta, R., Hirschmann, M. M. & Smith, N. D. (2007). Water follows carbon: CO2 incites deep silicate melting and dehydration beneath mid-ocean ridges. Geology 35, 135-138.
-
Donnelly, K. E., Goldstein, S. L., Langmuir C. H. & Spiegelman M. (2004). Origin of enriched ocean ridge basalts and implications for mantle dynamics. Earth and Planetary Science Letters 226, 347–366.
-
Downes, H., Beard, A. & Hinton, R. (2004). Natural experimental charges: an ion-microprobe study of trace element distribution coefficients in glass-rich hornblendite and clinopyroxenite xenoliths. Lithos 75, 1-17.
-
Downes, H. (2007) Origin and significance of spinel and garnet pyroxenites in the shallow lithospheric mantle: Ultramafic massifs in orogenic belts in Western Europe and NW Africa. Lithos 99, 1-24.
-
Gast, P. W. (1968). Trace element fractionation and origin of tholeiitic and alkaline magma types. Geochimica et Cosmochimica Acta 32, 1057-1086.
-
Gast, P. W., Tilton, G. R. & Hedge, C. (1964). Isotopic composition of lead and strontium from Ascension and Gough Islands. Science 145, 1181-1185.
-
Granet, M., Wilson, M. & Achauer, U. (1995). Imaging a mantle plume beneath the French Massif Central. Earth and Planetary Science Letters 136, 281-296.
-
Halliday, A. N., Davidson, J. P., Holden, P., Dewolf, C., Lee, D. C. & Fitton, J. G. (1990). Trace-element fractionation in plumes and the origin of HIMU mantle beneath the Cameroon line. Nature 347, 523-528.
-
Halliday, A. N., Davies, G. R., Lee, D. C., Tommasini, S., Paslick, C. R., Fitton, J. G. & James, D. E. (1992). Lead isotope evidence for young trace-element enrichment in the oceanic upper mantle. Nature 359, 623-627.
-
Halliday, A. N., Lee, D. C., Tommasini, S., Davies, G. R., Paslick, C. R., Fitton, J. G. & James, D. E. (1995). Incompatible trace-elements in OIB and MORB and source enrichment in the sub-oceanic mantle. Earth and Planetary Science Letters 133, 379-395.
-
Hart, S. R. (1988). Heterogeneous mantle domains—signature, genesis and mixing chronologies. Earth and Planetary Science Letters 90, 273-296.
-
Harte, B., Hunter, R. H. & Kinny, P. D. (1993). Melt geometry, movement and crystallization, in relation to mantle dykes, veins and metasomatism. Philosophical transaction of the royal society of London, Series A 342, 1-21.
-
Hoernle, K., Zhang, Y. S. & Graham, D. (1995). Seismic and geochemical evidence for large-scale mantle upwelling beneath the eastern Atlantic and western and central-Europe. Nature 374, 34-39.
-
Hofmann, A. W. & White, W. M. (1982). Mantle plumes from ancient oceanic-crust. Earth and Planetary Science Letters 57, 421-436.
-
Ionov, D. A. & Hofmann, A. W. (1995). Nb-Ta-rich mantle amphiboles and micas - Implications for subduction-related metasomatic trace-element fractionations. Earth and Planetary Science Letters 131, 341-356.
-
Kogiso, T., Hirschmann, M. M. & Reiners, P. W. (2004). Length scales of mantle heterogeneities and their relationship to ocean island basalt geochemistry. Geochimica et Cosmochimica Acta 68, 345-360.
-
Lloyd, F. E. & Bailey, D. K. (1975). Light element metasomatism of the continental mantle: the evidence and the consequences. Physics and Chemistry of the Earth 9, 389-416.
-
McDonough, W. F. & Sun, S. S. (1995). The composition of the Earth. Chemical Geology 120, 223-253.
-
McKenzie, D. (1989). Some remarks on the movement of small melt fractions in the mantle. Journal of Petrology 95, 53-72.
-
McKenzie, D. & O'Nions, R. K. (1995). The source regions of ocean island basalts. Journal of Petrology 36, 133-159.
-
McPherson, E., Thirlwall, M. F., Parkinson, I. J., Menzies, M. A., Bodinier, J.-L., Woodland, A. & Bussod, G. (1996). Geochemistry of metasomatism adjacent to amphibole-bearing veins in the Lherz peridotite massif. Chemical Geology 131, 135-157.
-
Moine, B. N., Gregoire, M., O'Reilly, S. Y., Sheppard, S. M. F. & Cottin, J. Y. (2001). High field strength element fractionation in the upper mantle: evidence from amphibole-rich composite mantle xenoliths from the Kerguelen Islands (Indian Ocean). Journal of Petrology 42, 2145-2167.
-
Morris, E. M. & Pasteris, J. D. (1987). Mantle Metasomatism and Alkaline Magmatism. Boulder: Geological Society of America, Special Paper 215, pp 383.
-
Nakamura, Y. & Tatsumoto, M. (1988). Pb, Nd, and Sr isotopic evidence for a multicomponent source for rocks of cook-Austral islands and heterogeneities of mantle plumes. Geochimica et Cosmochimica Acta 52, 2909-2924.
-
Nielson, J. E. & Noller, J. S. (1987). Processes of mantle metasomatism. In: E. M. Morris and J. D. Pasteris (eds.) Mantle Metasomatism and Alkaline Magmatism. Geological Society of America, Special Paper 215, 61-76.
-
Nielson, J. E. & Wilshire, H. G. (1993). Magma transport and metasomatism in the mantle: a critical review of current geochemical models. American Mineralogist 78, 1117-1134.
-
Niu, Y. L. & O'Hara, M. J. (2003). Origin of ocean island basalts: A new perspective from petrology, geochemistry, and mineral physics considerations. Journal of Geophysical Research-Solid Earth 108, B4, doi:10.1029/2002JB002048.
-
Palacz, Z. A. & Saunders, A. D. (1986). Coupled trace-element and isotope enrichment in the Cook-Austral-Samoa islands, southwest Pacific. Earth and Planetary Science Letters 79, 270-280.
-
Panter, K. S., Blusztajn, J., Hart, S. R., Kyle, P. R., Esser, R. & McIntosh, W. C. (2006). The origin of HIMU in the SW Pacific: Evidence from intraplate volcanism in southern New Zealand and subantarctic islands. Journal of Petrology 47, 1673-1704.
-
Pilet, S., Baker, M. B. & Stolper, E. M. (2008). Metasomatized lithosphere and the origin of alkaline lavas. Science 320, 916-919.
-
Pilet, S., Hernandez, J., Bussy, F. & Sylvester, P. J. (2004). Short-term metasomatic control of Nb/Th ratios in the mantle sources of intraplate basalts. Geology 32, 113-116.
-
Pilet, S., Hernandez, J., Sylvester, P. & Poujol, M. (2005). The metasomatic alternative for ocean island basalt chemical heterogeneity. Earth and Planetary Science Letters 236, 148-166.
-
Pilet, S., Ulmer, P. & Villiger, S. (2010). Liquid line of descent of a basanitic liquid at 1.5 GPa: Constrains on the formation of metasomatic veins. Contributions to Mineralogy and Petrology 159,621–643.
-
Prytulak, J. & Elliott, T. (2007). TiO2 enrichment in ocean island basalts. Earth and Planetary Science Letters 263, 388-403.
-
Shaw, A. M., Behn, M. D., Humphris, S. E., Sohn, R. A. & Gregg, P. M. (2010) Deep pooling of low degree melts and volatile fluxes at the 85 degrees E segment of the Gakkel Ridge: Evidence from olivine-hosted melt inclusions and glasses. Earth and Planetary Science Letters 289, 311-322.
-
Sun, S. S. & McDonough, W. F. (1989). Chemical and isotopic systematics of oceanic basalts; implications for mantle composition and processes. In: Saunders, A. D. & Norry, M. J. (eds.) Magmatism in the Ocean Basins: Geological Society of America, Special Paper 42, 313-345.
-
Thompson, R. N., Ottley, C. J., Smith, P. M., Pearson, D. G., Dickin, A. P., Morrison, M. A., Leat, P. T. & Gibson, S. A. (2005). Source of the quaternary alkalic basalts, picrites and basanites of the Potrillo Volcanic Field, New Mexico, USA: Lithosphere or convecting mantle? Journal of Petrology 46, 1603-1643.
-
Vaselli, O., Downes, H., Thirlwall, M., Dobosi, G., Coradossi, N., Seghedi, I., Szakacs, A. & Vannucci, R. (1995). Ultramafic xenoliths in Pliopleistocene alkali basalts from the eastern Transylvanian basin—Depleted mantle enriched by vein metasomatism. Journal of Petrology 36, 23-53.
-
Wass, S. Y. & Rogers, N. W. (1980). Mantle metasomatism—Precursor to continental alkaline volcanism. Geochimica et Cosmochimica Acta 44, 1811-1823.
-
Weaver, B. L. (1991). The origin of ocean island basalt end-member compositions - Trace-element and isotopic constraints. Earth and Planetary Science Letters 104, 381-397.
-
Wilshire, H. G. (1987). A model of mantle metasomatism. In: Morris, E. M. & Pasteris, J. D. (eds.) Mantle Metasomatism and Alkaline Magmatism: Geological Society of America, Special Paper 215, 47-60.
-
Wilson M. & Patterson R. (2001) Intraplate magmatism related to short-wavelengh convective instabilities in the upper mantle: Evidence from the Tertiary-Quaternary volcanic province of western and central Europe. In Ernst R. E. & Buchan K. L. (eds) Mantle Plumes: Their Identification Through Time: Geological Society of America Special Paper 352, 37-58.
-
Workman, R. K. & Hart, S. R. (2005). Major and trace element composition of the depleted MORB mantle (DMM). Earth and Planetary Science Letters 231, 53-72.
-
Workman, R. K., Hart, S. R., Jackson, M., Regelous, M., Farley, K. A., Blusztajn, J., Kurz, M. & Staudigel, H. (2004). Recycled metasomatized lithosphere as the origin of the enriched mantle II (EM2) end-member: Evidence from the Samoan volcanic chain. Geochemistry Geophsics Geosystems 5, doi:10.1029/2003GC000623.
-
Wulff-Pedersen, E., Neumann, E. R., Vannucci, R., Bottazzi, P. & Ottolini, L. (1999). Silicic melts produced by reaction between peridotite and infiltrating basaltic melts: ion probe data on glasses and minerals in veined xenoliths from La Palma, Canary Islands. Contributions to Mineralogy and Petrology 137, 59-82.
-
Wyllie, P. J. (1988a). Magma genesis, plate-tectonics, and chemical differentiation of the Earth. Reviews of Geophysics 26, 370-404.
-
Wyllie, P. J. (1988b). Solidus curves, mantle plumes, and magma generation beneath Hawaii. Journal of Geophysical Research - Solid Earth and Planets 93, 4171-4181.
-
Zanetti, A., Vannucci, R., Bottazzi, P., Oberti, R. & Ottolini, L. (1996). Infiltration metasomatism at Lherz as monitored by systematic ion-microprobe investigations close to a hornblendite vein. Chemical Geology 134, 113-133.
-
Zindler, A. & Hart, S. (1986). Chemical geodynamics. Annual Review of Earth and Planetary Sciences 14, 493-571.
last updated 21th
March, 2015 |