 |
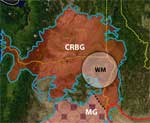 |
Delamination
Origin for Columbia River Flood Basalts and Wallowa
Mountains Uplift |
|
David
L. Abt1,2, T.
C. Hales1, Eugene
D. Humphreys1, & Joshua
J. Roering1
1Department
of Geological Sciences, University of Oregon, Eugene,
OR 97405 USA
2Now at Department
of Geological Sciences, Brown University, Providence,
RI 02906 USA
Contact: David L. Abt
Email: David_Abt@brown.edu
Phone: (401) 863-3339
Fax: (401) 863-2058
|
Read more
about this topic in a recent publication in the journal
Nature ( Hales
et al.,
2005).
Click here to
download a PDF version of this webpage
|
Introduction
The mechanism driving
flood basalt volcanism (i.e., hot spot initiation)
is undoubtedly an issue of great contention in many
geologic circles, and rightly so. These enormous events
represent large scale melting and, as many have argued,
may result from deep-reaching convective currents that
transport hot, fertile mantle to the surface (Morgan,
1972; Crough, 1983; Hill et al., 1992;
van Keken, 1997). However, our recent investigations
show that a plume-like mantle upwelling is not the principle
cause of Columbia River Basalt Group (CRBG) volcanism
in northeast Oregon, southwest Washington, and western
Idaho. The pattern of uplift observed in the region
is inconsistent with that expected from the impingement
of a rising plume head (e.g., Ribe &
Christensen, 1994; Farnetani
& Richards,
1994), but the upper mantle seismic structure is
consistent with it being the source region for CRBG
melts. Here we not only provide evidence supporting
our proposed model, but also hope to stimulate further
work on this important problem.
The northwestern United
States provides an ideal locale for the study of large
igneous provinces (Figure 1) because it is home to the
most recent flood basalt event on Earth, the effects
remain relatively unaltered by subsequent tectonic,
volcanic or erosional events, and an approximately plate-motion-parallel
progression of volcanism can easily be traced from southeast
Oregon across the Snake River Plain to Yellowstone.
The effects in northeastern Oregon include the eruption
of ~175,000 km3 of dominantly tholeiitic
basalt (Tolan et al., 1989), 300 m of syn-
and post-eruptive uplift over a broad region, and persistent
uplift of ~2 km in the Wallowa Mountains. It should
be noted that recent revisions to the classification
of flood basalt volcanism in the region have been made
bringing the total volume to ~234,000 km3
(Camp & Ross, 2004); the Columbia River
flood basalt province now includes not only the CRBG
but also the Steens and Malheur Gorge basalts of southeastern
Oregon. Though it is likely they are closely related,
our study focuses on the source of volcanism and uplift
in northeastern Oregon rather than the entire province.
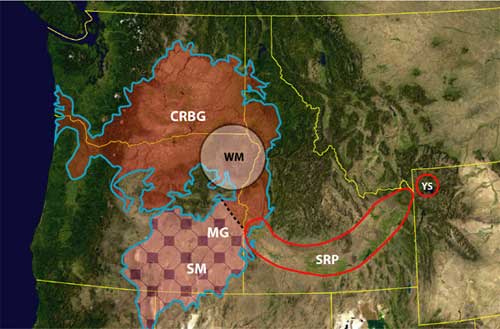
Figure 1. Map showing
the distribution of the mid-Miocene Columbia River flood
basalts (CRBs). The white circle denotes the region
of uplift described in Figure 2. We separate the CRBs
into the CRBG and Steens/Malheur Gorge basalts because
of their distinct source regions. MG = Malheur Gorge,
SM = Steens Mountain, SRP = Snake River Plain, WM =
Wallowa Mountains, YS = Yellowstone.
Regional Uplift
(Mid-Miocene to Present)
CRBG lavas erupted onto
a low relief surface of Mesozoic accreted oceanic terranes
stitched together by Jurassic plutons, of which the
Wallowa pluton is the largest (Goles, 1986).
After initial Imnaha flows filled valleys, subsequent
Grande Ronde (GR) eruptions deposited thin, flat-lying
sheets. The well-mapped, large, continuous areal extent
of these early CRBG flows allows for detailed flow interface
analysis to accurately measure deformation (Figure 2a).
We correct elevation maps of the GR magnetostratigraphic
units (Walker, 1979; Hooper et al.,
1992; Reidel et al., 1995) for the effects
of erosional unloading (Lambeck, 1988; Anderson,
1994) to obtain markers of post-eruptive deformation.
Imnaha basalts ponded in incipient basins, indicating
possible pre-eruptive subsidence (Camp, 1981).
Basin development continued during GR eruptions, causing
local ponding and thickening of flows, while syn-eruptive
uplift created locally thinned units. Post-GR deformation
is evidenced by the contrasting distributions of GR
and later CRBG Wanapum (15-14 Ma) and Saddle Mountains
(14-6 Ma) flows, which show distinctive, well-developed
channelization and ponding in response to emerging topographic
relief (Tolan et al., 1989). The uplift and
downwarp of these flows closely conform to current topography,
with up to 2 km of uplift focused on the Wallowa pluton
(Figure 2b) and more subdued uplift centred on the lesser
plutons in the area. This structural evidence leads
us to conclude that most Wallowa Mountains uplift occurred
after the majority of CRBG eruptions and over a period
of <10 m.y, and we find the volume of uplift relative
to the hinge-line in Figure 2b is ~7300 km3.
|
|
Figure
2. Post-eruptive uplift. (a) Digital elevation
map showing the overlapping distribution of exposed
GR magnetostratigraphic interfaces: Imnaha-R1
(blue), R1-N1 (green), N1-R2 (gold) and R2-N2
(red). (b) Composite surface for GR uplift. This
surface was made by vertically shifting the individual
interfaces using published flow thicknesses (Camp,
1981), smoothing the resulting surface with a
mild low-pass filter, and removing the effects
of erosional unloading by deconvolving the point
load response of an elastic plate (Lambeck, 1988;
Anderson, 1994), assuming total coverage by the
GR lavas and an elastic thickness of 5 km, similar
to other estimates from this area (Lowry &
Smith, 1995). The absolute elevation is referenced
to a hinge line (black line) that separates the
uplifted Blue Mountains from the down-dropped
Pasco Basin immediately NW of the Blue Mountains. |
Upper Mantle
Seismic Structure
We have also conducted
the first tomographic study of the upper mantle in northeast
Oregon using a six-station array (Figure 3a). Lateral
resolution at shallow depth is low due to large station
spacing (75-150 km), but the broad aperture of the network
allows for reasonable imaging to depths of 250 km. There
exists a high-velocity region between ~70 km and ~150
km depth centered beneath the Wallowas and other granite-cored
mountains in the region (Figure 3b). “Squeezing”
tests indicate no need for significant anomalies below
~175 km. Other less-well-resolved structures include
two separate high-velocity bodies: (1) beneath station
IDA in western Idaho at shallow depth and (2) deeper
structure beneath the western Snake River Plain. The
remaining upper mantle imaged indicates slower than
normal (relative to IASPI91) seismic velocities. Maximum
deviations in Vp are ±4%.
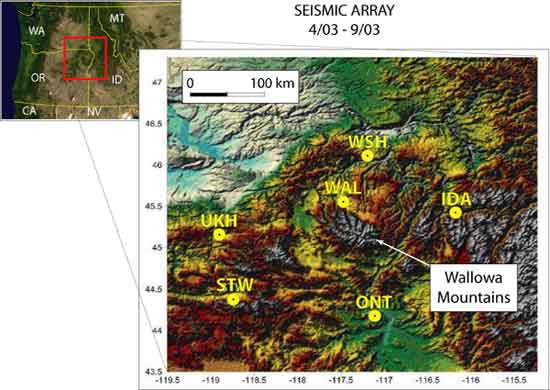
Figure
3a
|
Figure
3. (a) Study area and seismic array utilized for
the tomographic inversion. Sensors were all Guralp
CMG40-T broadband seismometers from PASSCAL. (b)
Upper mantle seismic structure from ~600 teleseismic
rays. The largest and best-resolved structure
is the high-velocity anomaly centered beneath
station WAL and the Wallowa Mountains. The rough
dimensions of this body are 80 km thick, 125 km
N-S, and 200 km E-W. The shallow structure beneath
IDA is at sub-crustal depth, and if real, could
represent residuum in the spinel-peridotite stability
field where an increase in seismic velocity is
expected (Schutt & Lesher, 2005) without a
significant density decrease. The high-velocity
anomaly southeast of ONT may be related to volcanism
in the western SRP or MG/SM basalts. Without a
broader array, the extent of these features remains
ambiguous. The remaining low-velocity mantle is
likely a result of excess heat or partial melt
from the flood basalt event. |
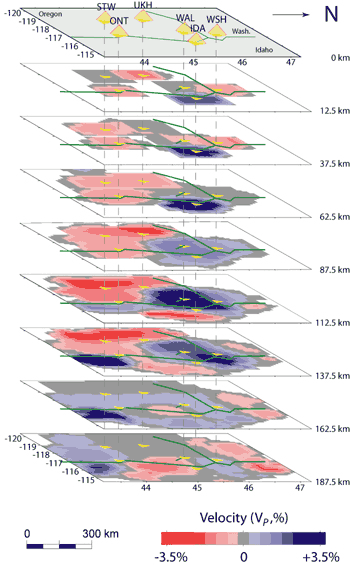
Figure
3b |
Discussion
Schutt and Lesher
(2005) investigated the effects of partial melting on
mantle peridotite residuum density and seismic velocity.
Though they find a density decrease of 0.65% per 10%
partial melting in the garnet stability field (i.e.,
below 70 km), no significant perturbations in Vp
, Vs, or Vp/Vs
are observed. Thus, if our imaged body is residuum from
the CRBG, a different mechanism for increasing seismic
velocity is needed. The occurrence of basaltic volcanism
in the recent past and the major uplift in the Wallowa
Mountains are inconsistent with the high-velocity mantle
being relatively cool. We argue that a reasonable alternative
is that melt content has been reduced (and possibly
eliminated) by eruption of the CRBG to the extent that
seismic velocity is increased. The hypothesis that this
anomaly is in fact the source region for the CRBG is
strengthened by the spatial correlation between the
upper mantle high-velocity volume and the location of
flood basalt dikes. Also, high pressure melting experiments
on a member of the GR basalts (GR make up 85% of the
CRBG) indicate a melting depth of ~70 km (Takahashi
et al., 1998), coincident with the upper limit
of the imaged high-velocity body.
If we assume that all
melt produced here was erupted (i.e., the volume
of the CRBG) [1.75 x 105 km3]
and conservatively estimate the volume of mantle source
region (our high-velocity body) [2 x 106
km3], then we can find an approximation
for the amount of partial melting needed to produce
the CRBG [6.84%]. Using this percentage,
an estimate of positive mantle buoyancy [2.64
x 103 kg] is possible, and we can
compare this with the volume of uplifted crust [7.3
x 103 km3] and its negative
buoyancy [2 x 103 kg]. These
simple (very rough) calculations demonstrate that the
high-velocity volume of mantle imaged is a very good
candidate for the source of the CRBG as well as the
isostatic support for the syn- and post-eruptive uplift
in the region.
Several plume-related
theories have been proposed for the origin of the CRBG.
For example, northward deflection of a plume by the
subducting Juan de Fuca slab (Geist & Richards,
1993), sub-lithospheric melting beneath southeast Oregon
with melt then transported laterally through the crust
some 400 km north (Takahashi et al., 1998,
see their figure 8), and radiating volcanic migrations
from a plume in southeast Oregon (Camp & Ross,
2004; Ed: see also Radiating
Volcanic Migrations & Columbia
River Basalts & Yellowstone
pages). However, none are able to account for the combined
pattern of uplift and seismic structure.
The formation of Rayleigh-Taylor
instabilities and subsequent mechanical detachment of
sections of the lithosphere has been suggested as the
cause for the Siberian flood basalts (Elkins-Tanton
& Hager, 2000). Delamination of lithospheric
material allows for the rapid upwelling of hot (and
likely fertile) asthenosphere to unusually shallow depths
where it can then readily melt through decompression.
The catalyst in their model is the intrusion of relatively
small amounts of dense adiabatic melts into the lower
lithosphere causing it to weaken and mechanically decouple
from the upper lithosphere. Unlike the Pacific Northwest,
Siberia does not show evidence for significant uplift
following the rapid eruption of flood basalts, but it
is likely that lithospheric structure plays a role in
determining the pattern of uplift in a different provinces
(Burov
& Guillou-Frottier,
2005). The extensive volcanic and tectonic history
of the accreted terranes in northeastern Oregon must
certainly have had an effect here; possibly by (1) creating
a weak layer within the lithosphere that would later
facilitate a delamination event and (2) forming a density
structure conducive to uplift after delamination (e.g.,
buoyant plutons with dense roots). Prior to the physical
separation of the lower lithosphere, uplift forces,
either from a thermal anomaly or buoyant residuum, would
have been counteracted by the downward pull of detaching
material. Following delamination, extensive upwelling
and melting formed the GR basalts, and the buoyant residuum
that today supports the regional uplift was emplaced.
Summary
With our new tomographic
images and uplift analysis, we believe that delamination
of dense mantle lithosphere in northeast Oregon ultimately
produced the CRBG and subsequent uplift of the region.
It remains unclear, however, what controlled the timing
and location of this event. Did the pre-existing lithospheric
structure coincidentally fail at the same time as the
impingement of a plume head 400 km to the south? Did
delamination occur (e.g., Elkins-Tanton
& Hager, 2000), triggered by melt intrusion
from a plume head spreading north from southeast Oregon?
Did the Precambrian margin to the east play a role in
creating flow from beneath the older, thicker lithosphere
up to the accreted Mesozoic terranes without the help
of a plume (e.g., King
& Anderson,
1998)? These are only a few of the questions that
remain unanswered, but with this and future, higher-resolution
tomographic studies we have a new means of addressing
and resolving the origin of the CRBs and possibly other
flood basalt eruptions.
|
References
-
Anderson, R. S.
(1994), Evolution of the Santa Cruz Mountains, California,
through tectonic growth and geomorphic decay.
J. Geophys. Res. 99, 20161-20179.
-
-
Camp, V. E. (1981),
Geologic studies of the Columbia Plateau: Part II.
Upper Miocene basalt distribution, reflecting source
locations, tectonism, and drainage history in the
Clearwater embayment Idaho. GSA Bull. 92,
669-678.
-
Camp, V. E. &
Ross, M. E. (2004), Mantle dynamics and genesis
of mafic magmatism in the intermontane Pacific Northwest.
J. Geophys. Res. 109, doi:10.1029/2003JB002838.
-
Crough, S. T. (1983),
Hotspot swells, Ann. Rev. Earth Planet. Sci.
Lett. 11, 165-193.
-
Elkins-Tanton,
L. T, and Hager, B. H. (2000), Melt intrusion as
a trigger for lithospheric foundering and the eruption
of the Siberian flood basalts, Geophys. Res.
Lett. 27, 3937-3940.
-
-
Geist, D., and
Richards, M. (1993), Origin of the Columbia Plateau
and Snake River Plain: deflection of the Yellowstone
plume, Geology 21, 789-792.
-
Goles, G. G. (1986),
Miocene Basalts of the Blue Mountains Province in
Oregon. I: Compositional Types and their Geological
Settings. J. Petrology 27,
495-520.
- Hales,
T.C., D. L. Abt, E. D. Humphreys and J. J. Roering,
Lithospheric instability origin for Columbia River
flood basalts and Wallowa Mountains uplift in northeast
Oregon, Nature, 438, 842-8452005.
-
Hill, R. I., Campbell,
I. H., Davies, G. F., and Griffiths, R. W. (1992),
Mantle plumes and continental tectonics, Science
256, 186-193.
-
Hooper, P. R.,
Webster, G. D. & Camp, V. E. (1985), Geologic
Map of the Clarkson 15 Minute Quadrangle, Idaho
and Washington. State of Washington Department
of Natural Resources Geologic Map Series GM-31.
-
King, S. D., and
Anderson, D. L. (1998), Edge-driven
convection, Earth Planet. Sci. Lett.
160, 289-296.
-
Lambeck, K. Geophysical
Geodesy, the Slow Deformations of the Earth
(Clarendon Press, Oxford, 1988).
-
Lowry, A. R., and
Smith, R. B. (1995) Strength and rheology of the
western U.S. Cordillera, J.Geophys. Res.
100, 17,947-17,963.
-
Morgan, W.J. (1972),
Plate motions and deep mantle convection, Mem.
Geo. Soc. Am. 132, 7-22.
-
Ribe, N. M., and
Christensen, U. R. (1994), Three-dimensional modeling
of plume-lithosphere interaction, J. Geophys.
Res. 99, 669-682.
-
Reidel, S. P.,
Hooper, P. R., Webster, G. D. & Camp, V. E.
(Washington Division of Geology and Earth Resources,
1992).
-
Reidel, S. P. et
al. (1989), The Grande Ronde Basalt, Columbia River
Basalt Group; Stratigraphic descriptions and correlations
in Washington, Oregon, and Idaho. Geological
Society of America Special Paper 239, 21-54.
-
Schutt, D. L.,
and Lesher, C. E. (2005), The effect and seismic
velocity of garnet and spinel lherzolite, J.
Geophys. Res., in press.
-
Tolan, T. L. et
al. (1989), Revisions to the estimates of the areal
extent and volume of the Columbia River Basalt Group.
GSA Special Paper 239, 1-20.
-
Takahashi, E., Nakajima,
K., and Wright, T. L. (1998), Origin of the Columbia
River basalts: melting model of a heterogeneous
plume head, Earth Planet. Sci. Lett. 162,
63-80.
-
van Keken, P. (1997),
Evolution of starting mantle plumes: a comparison
between numerical and laboratory models, Earth
Planet. Sci. Lett. 148, 1-11.
-
Walker, G. W. (1979),
Reconnaissance Geologic Map of the Oregon Part of
the Grangeville Quadrangle, Baker, Union, Umatilla,
and Wallowa Counties, Oregon. USGS Miscellaneous
Investigations Series, Map I-1116.
|
last updated 20th
December, 2005 |
|
|