 |
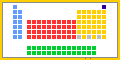 |
Helium:
Fundamental models |
|
Don
L. Anderson1, G.
R. Foulger2 & Anders
Meibom3
1Seismological
Laboratory, California Institute of Technology,
Pasadena, CA 91125
dla@gps.caltech.edu
2Dept.
Geological Sciences, University of Durham, Durham
DH1 3LE, U.K.
g.r.foulger@durham.ac.uk
3Laboratoire
d'Etude de la Matiere Extraterrestre, USM 205
(LEME), Case Postale 52, Museum National d'Histoire
Naturelle, 61 rue Buffon, 75005 Paris
meibom@mnhn.fr |
Abstract
Helium is rare in the Earth but
has become an important geochemical tracer since is
it widely considered to be the only unambiguous geochemical
indicator of a lower mantle and plume component in
surface rocks. In practice, observations of 3He/4He
higher than ~ 10 times the atmospheric value are generally
interpreted as evidence for a plume from the lower
mantle, even in the absence of supporting data. This,
however, is strictly an assumption.
There is evidence that high-3He/4He
may originate in the upper mantle. This model is more
consistent with other observations at “hotspots”
where a shallow-mantle origin is strongly supported
and suggests that high-3He/4He
cannot be used to prospect for plumes.
|
3He
is a so-called primordial isotope. It was made
in the Big Bang and incorporated into Earth
during its initial accretion and in the subsequent
long-term acquisition of “late veneer”
material. 3He is not produced in
any large quantities by radiogenic decay, and
is thus not being added to Earth’s inventory
at a significant rate. Nevertheless, a small
amount is constantly being added to the surface
of the Earth by interplanetary dust particles
[Anderson, 1993] and by cosmic rays.
This so-called “cosmogenic” 3He
may be important in rocks that have lain at
the surface of the Earth for long periods. |
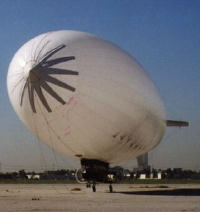
Figure 1: Industrial
helium is mostly 4He and is mined
from helium mines |
4He is a product of alpha
decay of U and Th, and accumulates over time. This
accumulation is most rapid in rocks that are rich
in U+Th, but may be very slow in rocks that contain
little U+Th. The U+Th content of mantle rocks and
recycled material varies by 3 or 4 orders of magnitude
and the opportunity therefore arises to develop large
variations in 3He/4He ratios.
The Earth is constantly degassing, which
transports helium from the crust and mantle into the
oceans and atmosphere. Because it is such a light
atom, helium escapes from Earth and is thereby continually
lost from the atmosphere. The approximate lifetime
of helium in the atmosphere is ~ 1 to 2 Myr.
The absolute abundance of helium in
rocks is difficult to interpret since helium is so
mobile. Thus, the 3He/4He ratio
(R) is usually used as a proxy for 3He
content. R is generally expressed as a multiple of
the present-day atmospheric 3He/4He
ratio, Ra, which is 1.38 x 10-6.
|
The average
value of 3He/4He in
Earth when it originally formed may have been
~ 200 Ra, but it has decreased subsequently
as a result of the addition of 4He
by U+Th decay over the 4.5-billion-year lifetime
of the planet. The amount by which it has
decreased in a given rock sample is related
to the integrated U+Th content of the sample
and, importantly, the absolute abundance of
helium. For example, if 4He is
added at a given rate to a sample rich in
helium, the value of 3He/4He
will change only slowly. On the other hand,
if the sample is poor in helium, its 3He/4He
ratio will decrease rapidly. Because rocks
in Earth are continually and variably experiencing
degassing and metasomatism (which may involve
the addition of gas), even if U+Th is known,
the rate of change of 3He/4He
with time cannot be calculated accurately.
The ratio in the atmosphere is also constantly
changing because it represents a balance between
extra terrestrial input, degassing and atmospheric
escape.
|
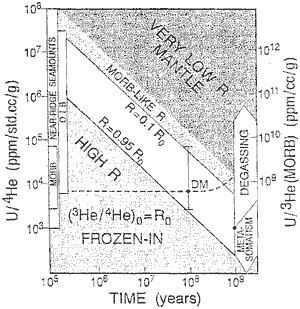
Figure 2: Trade-off curves
between U/4He and time, which give
high and low 3He/4He
reservoirs [from Anderson,
1998b].
|
The observed value
of 3He/4He varies in terrestrial
rocks. Some typical values for R/Ra are:
- Continental rocks (high-U+Th)
<< 1
- Commonly assumed for mid-ocean
ridge basalt (MORB) 8 ± 2
- Average spreading ridge basalt
9.1 ± 3.6
- Ocean island basalt (OIB) (“hotspot”
rocks) ~ 5 - 42
The highest, non-cosmogenic
value for R/Ra reported for “hotspot”
rocks anywhere on Earth (42) is from Iceland [Breddam
& Kurz, 2001].
|
The standard model
Values for 3He/4He
greatly exceeding those typical of MORB were first
observed in some basalts from Hawaii. It was suggested
that they were indicative of a lower mantle component,
since Hawaii was postulated to be underlain by a plume,
and plumes were assumed to originate in the lower
mantle to explain the apparent fixity of hotspots
[Morgan, 1971].
It was thus concluded that the lower mantle is characterised
by high 3He/4He – perhaps
25 or 30 Ra. This number has been continually upward-adjusted
as higher and higher 3He/4He
values have been found, e.g., from Iceland, and in
ancient rocks such as flood basalts and komatiites.
A model for helium has
been developed with a depleted, degassed and homogenized
upper mantle, “the convecting mantle”,
and a lower mantle that is little- or undegassed,
and contains much more 3He than the upper
mantle. By this reasoning, the high 3He/4He
ratios were attributed to an abundance of 3He
in the “primordial undegassed” mantle.
This model is flawed
for a number of reasons:
1. Mass balance
calculations show that it predicts an unreasonably
high concentration of 3He, relative
to other volatiles and incompatible elements,
in the lower mantle [ Anderson,
1989]. This follows because over the lifetime
of Earth, radioactive decay of U+Th in the lower
mantle has generated a large amount of 4He.
In order for the value of 3He/ 4He
in the lower mantle to have remained high, the
concentration of 3He there must be
high. Calculations show that it is predicted
to be about an order of magnitude less than
that in chondritic meteorites, relative to refractory
elements [ Kellogg & Wasserburg,
1990]. This is contrary to expectations that
it should be several orders of magnitude
less (see Figure at right). The problem of the
high predicted relative concentration of 3He
in the lower mantle becomes greater as the maximum
3He/ 4He observed at the
surface increases. Most recently, a value of
3He/ 4He > 50 Ra was
reported from Baffin Island [Stuart, 2003],
which is far higher than the value of ~ 30 Ra
assumed by Kellogg & Wasserburg
[1990]. |
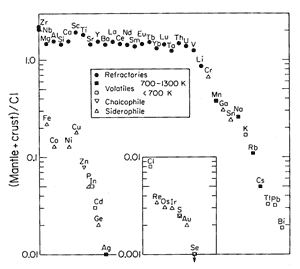
Figure 3: Abundances
of elements in the crust+mantle relative to
those in chondritic meteorites (from Anderson,
1989; Figure 8.2) |
A high abundance of
[3He] in the lower mantle is also at odds
with high-temperature models of planetary accretion.
The Earth is thought to have accreted from meteoric
material, but subsequently huge amounts of heat were
generated by gravitational compaction, segregation
of the core, and impact with a Mars-sized body that
caused the Moon to form. The Earth thus went through
one or more phases where it was largely or completely
molten. Extensive degassing would have occurred at
these times, which is supported by the observation
that Earth is strongly depleted in volatiles such
as Na, K, Cl, CO2, H2O, and
Rb (see Figure). By this reasoning, a model whereby
a large part of Earth is undegassed is unlikely to
be right. Furthermore, mass balance calculations show
that about 70% of the mantle must be depleted in the
volatile and crust-forming elements, and this is consistent
with the amount of 40Ar in the atmosphere
[Anderson,
1989]. The volume above the 650 km discontinuity,
the usual choice for the depth to the top of the depleted,
undegassed reservoir, is only 30% of the mantle. This
choice therefore cannot be correct, since most of
the mantle must have contributed to the incompatible
elements in the crust and the 40Ar in the
atmosphere.
2. If the concentration
of helium in the lower mantle is very large –
much larger than in the upper mantle, then helium
abundances in OIB are predicted to be much higher
than in MORB. This is not observed. The helium abundances
in OIB are 2 – 3 orders of magnitude less than
in MORB. It has been suggested that this is due to
preferential degassing of OIB as a result of their
shallow depth of eruption. This can be checked by
looking at the relative concentrations of the heavier
noble gases.
The
heavy noble gasses are expected to have a greater
tendency to degas upon eruption than helium,
so He/Ne, He/Ar etc. should be higher, the more
degassed a rock is. It is found that He/Ne and
He/Ar are lower in OIB than in MORB, however,
suggesting OIB is less degassed than MORB, not
more [ Moreira & Sarda, 2000; Ozima
& Igarashi, 2000]. This is supported
by CO 2:He systematics at Hawaii.
CO 2 is a helium carrier, and the
quantities of CO 2 being degassed
at Hawaii are not consistent with the source
having a high abundance of helium. Most of the
3He being degassed from the mantle
is from mid-ocean ridges and island arcs. The
model whereby OIB were originally high in helium,
which has been lost by degassing accompanying
eruption, is therefore not supported –
mid-ocean ridges exhale more 3He
than hotspots [ Anderson,
1998a; Anderson,
1998b]. |
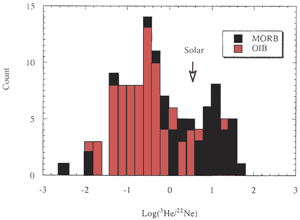
Figure 4: Histogram
of 3He/22Ne in MORB and
OIB. "Solar" indicates the cosmic
abundance ratio. MORB and OIB are fractionated
in opposite directions relative to the solar
ratio [from Ozima & Igarashi, 2000]. |
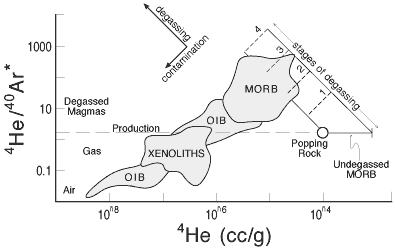 |
Figure
5: Plot showing relation between helium
and argon isotopes in MORB and OIB and the effects
of degassing and "contamination" with
air and older CO2-rich vesicles (Anderson,
2000a). |
|
An alternative model
In response to a growing body of observations
that support shallow sources for some high-3He/4He
hotspots, models that attribute an upper-mantle origin
to high 3He/4He have been developed.
It has been suggested that high 3He/4He
results from a deficit of 4He consequential
to storage of old helium in a low time-integrated
U+Th host rock [Anderson,
1998a; Anderson,
1998b]. The controlling parameters are then U/3He,
U/Th and time. There are several lines of evidence
that support this model:
a) high 3He/4He
is observed in Samoan xenoliths that are known to
be of upper mantle origin,
b) high 3He/4He
has been measured in diamonds known to have been mined
from pipes. (High 3He/4He has
been reported in diamonds of unknown origin, but in
these cases it is has been suggested that they may
be “detrital” diamonds, i.e.,
they may have lain on the surface for a long time
and acquired “cosmogenic” 3He.
Although this is conjecture, it is safer to use diamonds
known to be from pipes.)
c) high 3He/4He
is observed at Yellowstone, where extensive work has
provided a strong case that the magmatic system there
is lithospheric only [see Yellowstone
page & Christiansen
et al.,
2002].
A possible low-U+Th host material is
the residuum left after basalt melt is extracted from
mantle peridotite [e.g., Brooker
et al., 2003]. Residuum contains only traces
of U+Th, and thus helium stored for hundreds of millions
of years would preserve its older, higher 3He/4He
ratio little changed [Anderson,
1998a; Anderson,
1998b]. One model for high 3He/4He
involves trapping of gases from ascending MORB magmas
in recycled oceanic lithosphere or olivine-rich cumulates.
Another possible host is individual
olivine crystals [Natland, 2003; Brooker
et al., 2003]. These grow so as to encapsulate
gas bubbles containing He, but olivine itself contains
essentially no U+Th. Alpha particles generated by
U+Th decay in surrounding minerals have insufficient
energy to penetrate olivine crystals, and thus the
encapsulated helium is effectively shielded from the
addition of 4He at the time of decay. Diffusion
of 4He probably has little effect also.
Solid state diffusion coefficients for most lithophile
elements (including U and Th) are of the order of
1013 cm2s-1 and even
on the time scale of the age of the Earth, this only
allows a characteristic length scale of diffusion
of the order of a few kilometers. On time scales less
than a few 100 My diffusion should thus have little
effect in domains of moderate size. Furthermore, diffusion
is driven by differences in chemical potential, not
simply by concentration gradients, and thus high partition
coefficients can block diffusion even in the presence
of large concentration gradients. He is volatile and
highly soluble in trapped CO2-rich bubbles
in olivine, but is essentially insoluble in olivine
itself. Thus, even though there might be a high concentration
of He in a trapped bubble inside an olivine crystal,
He will not tend to diffuse out. 4He would
have a greater tendency to diffuse into a trapped
bubble, but there would be little 4He present
in thick, olivine-rich cumulate lithologies from which
melt, and thus U+Th, had been squeezed out. Unradiogenic
He trapped in olivine crystals in this way will be
well protected from the addition of 4He,
even over long time scales.
This suggests a “He in olivine”
model, whereby noble gases are trapped in olivine
and pyroxene in a cumulate olivine-gabbroic layer
that forms the lowermost oceanic crust. This layer
comprises densely packed cumulates that have compacted
and squeezed out the interstitial melt, thereby expelling
essentially all the U+Th. It may be subducted, and
if young and warm will be buoyant and remain in the
upper mantle. When it is once more transported into
a melting region, it will produce voluminous, high-3He/4He
melts.
The resulting He isotope composition
of the melt will depend on the age and abundance of
the olivine-gabbroic material, how much helium is
trapped, and how much 4He is generated
in the surrounding rock. Stochastic sampling of such
a source would predict Gaussian distributions of 3He/4He
isotope ratios similar to those expected for MORB
but with more variability and no global correlation
with large-ion lithophile elements and isotope systems,
though local correlations may occur. This kind of
distribution is precisely what is observed [Meibom
et al., 2003].
A schematic diagram showing a model
for the evolution of helium isotope ratios in the
Earth is shown in Figure 6.
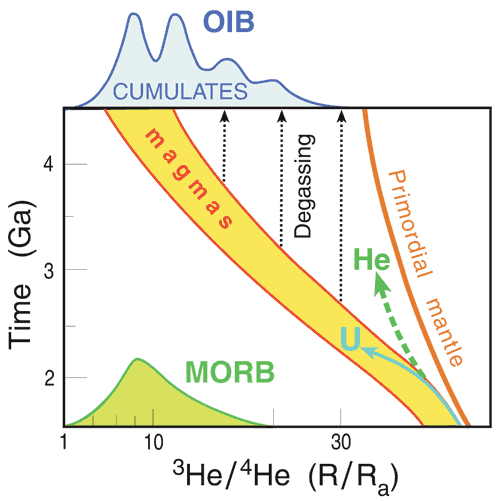
Figure 6: The Primordial
mantle curve shows the growth of 3He/4He
in a hypothetical primordial reservoir from Big Bang
values (right) to present (left). This is how the
lower mantle is predicted to have evolved in the Standard
Model. At various times magma is extracted (MAGMAS).
In this magma, or the lavas formed when it freezes,
3He/4He evolves faster because
U and Th were preferentially partitioned into the
magma and gas was lost to the atmosphere or retained
in the residual mantle. Simply put, differentiation
and degassing changes the 3He/(U,Th) ratio
of the products and resulting evolution of 3He/4He.
The magmas lose more gas as they rise to shallow depths,
decreasing the He/U ratio still further and increasing
the He/Ar and He/Ne ratios. Some of the gas lost ends
up in olivine-rich cumulates and gas inclusions in
peridotites, imparting to them relatively high He/U
and 3He/4He. Ancient 3He/4He
ratios may thus be frozen into whatever material the
gas ends up in, while the residual degassed magma
goes on to evolve to low values. The trajectories
of He (and CO2) and U (and Th) following
melting and degassing events are shown schematically.
Each melting/degassing event gives this kind of fractionation.
The dashed lines indicate schematically how ancient
3He/4He ratios are frozen in
as He is separated from U and Th and stored elsewhere.
The MORB and OIB
histograms are plotted at arbitrary positions on the
time axis but represent current values. The samples
that make up the OIB field represent a mix of magmas
and gases of various ages stored in the mantle. It
is closer to the actual mantle distribution than the
suite of values measured in MORB because OIB melt
batches are smaller than MORB batches. Large samples
of material with OIB-like 3He/4He
distributions, homogenised, will have a MORB-like
3He/4He distribution (Anderson,
2002b) because large-batch homogenising supresses
outliers. For example, the mantle today might contain
regions where R/Ra varies from, say, 1 to 100, but
the variation in MORB might be no more than 5 to 10
simply as a result of sampling and homogenising. OIB
extracted from the same mantle might have R/Ra values
that vary from, say, 2 to 30, as a result of the extraction
of smaller-volume samples.
|
Summary
The model whereby high 3He/4He
is attributed to a lower-mantle source, and is thus
effectively an indicator of plumes from the lower
mantle, is becoming increasingly untenable as evidence
for a shallow origin for many high-3He/4He
hotspots accumulates. Shallow, low-4He
models for high-3He/4He are
logically reasonable, cannot be ruled out, and need
to be rigorously tested if we are to understand the
full implications of this important geochemical tracer.
|
-
-
Anderson,
D.L., Helium-3 from the mantle: primordial signal
or cosmic dust?, Science, 261,
170-176, 1993.
-
-
-
- Anderson,
D. L., The Statistics of helium isotopes along the
global spreading ridge system and the Central Limit
Theorem, Geophys. Res. Lett., 27,
2401-2404, 2000b.
-
Breddam,
K., and M.D. Kurz, Helium isotope signatures of
Icelandic alkaline lavas, EOS Trans. AGU,
2001.
-
-
Kellogg,
L.H., and G.J. Wasserburg, The role of plumes in
mantle helium fluxes, Earth planet. Sci. Lett.,
99, 276-289, 1990.
-
Meibom,
A., D.L. Anderson, N.H. Sleep, R. Frei, C.P. Chamberlain,
M.T. Hren, and J.L. Wooden, Are high 3He/4He
ratios in oceanic basalts an indicator of deep-mantle
plume components?, Earth planet. Sci. Lett.,
208, 197-204, 2003.
-
Moreira,
M., and P. Sarda, Noble gas constraints on degassing
processes, Earth planet. Sci. Lett., 176,
375-386, 2000.
-
-
-
Ozima, M.,
and G. Igarashi, The primordial noble gases in the
Earth: a key constraint on Earth evolution models,
Earth planet. Sci. Lett., 176,
219-232, 2000.
- Stuart, F.M., S. Lass-Evans,
J.G. Fitton and R.M. Ellam, Extreme 3He/4He
in picritic basalts from Baffin Island: the role of
a mixed reservoir in mantle plumes, Nature,
in press, 2003.
and for those
who are really into all this.....
- Anderson, D.L., The statistics
of helium isotopes along the global spreading ridge
system and the central limit theorem, Geophys.
Res. Lett., 27, 2401-2404,
2000.
- Anderson, D.L., The statistics
and distribution of helium in the mantle, Int.
Geology Rev., 42, 289-311,
2000.
- Brooker,
R.A., V. Heber, S.P. Kelley and B.J. Wood, Noble
gas partitioning behaviour during mantle melting:
A possible explanation for “The He Paradox”?,
EOS Trans. AGU Fall Meet. Suppl., Abstract,
V31F-03, 2003.
- Dodson A., Kennedy B.M., and
DePaolo D.J., Helium and neon isotopes in the Imnaha
Basalt, Columbia River Basalt Group: Evidence for
a Yellowstone Plume Source, Earth Planet. Sci.
Lett.,150, pp. 443-451, 1997.
- Ellam, R.M. & F.M. Stuart,
Isotope geochemistry of the North Atlantic Igneous
Province: a Tertiary to Recent record of a mantle
plume, J. Petrol., 141,
919-932, 2000.
- Foulger,
G.R. and D.G. Pearson, Is Iceland underlain by a
plume in the lower mantle? Seismology and helium
isotopes, Geophys. J. Int. (Fast Track),
145, F1-F5, 2001.
- Graham, D. W., J. E. Lupton,
F. J. Spera & D. M. Christie, Upper mantle dynamics
revealed by helium isotope variations along the
Southeast Indian Ridge, Nature, 409,
701-703, 2001.
- Graham, D. W., Noble gas isotope
geochemistry of mid-ocean ridge and ocean island
basalts; characterization of mantle source
reservoirs. In: Noble Gases in Geochemistry
and Cosmochemistry, eds. D. Porcelli, R. Wieler
& C. Ballentine, Reviews in Mineralogy and Geochemistry,
Mineral. Soc. Amer., Washington, D.C., pp. 247-318,
2002.
- Hanan, B.B. & Graham, D.W.,
Lead and Helium Isotope Evidence from Oceanic Basalts
for a Common Deep Source of Mantle Plumes, Science
272, 991-995, 1996.
- Ozima, M. & F.Podosek,
Noble Gas Geochemistry, Cambridge University
Press, New York, 2002.
- Stuart, F.M., R.M. Ellam, P.J.
Harrop, J.G. Fitton & B.R. Bell, Constraints
on mantle plumes from the helium isotopic composition
of basalts from the British Tertiary igneous province,
Earth Planet. Sci. Lett., 177,
273-285, 2000.
Volcano noble gas database
A new USGS online database: USGS-NoGaDat
– A Global Dataset of Noble Gas Concentrations
and Their Isotopic Ratios in Volcanic Systems, by
A.A. Abedini, S. Hurwitz, and W.C. Evans, U.S. Geological
Survey DigitalData Series, 202. http://pubs.water.usgs.gov/ds202/
This database contains almost 5,000
entries of published information on noble gas concentrations
and isotopic ratios from volcanic systems in mid-ocean
ridges, ocean islands, seamounts, and oceanic and
continental arcs. Where available the isotopic ratios
of strontium, neodymium, and carbon were also included.
The database is sub-divided both into material sampled
(e.g., volcanic glass, different minerals,
fumarole, spring), and into different tectonic settings
(MOR, ocean islands, volcanic arcs). Included is also
a large reference list.
|
last
updated 2nd September, 2006 |
|
|