Hotspots
or no Hotspots?
Where do
hotspots come from? What is their source
depth? Do they provide a fixed reference
frame? Hotspot tracks have been used to
compute the motion of plates relative
to the mantle. For this purpose it is
fundamental to know whether hotspots are
i) fixed relative to the mantle, ii) fixed
relative to one another, and iii) from
what depth they originate. However hotspots
have often been used uncritically, without
regard to their real nature. Volcanic
tracks at the Earth's surface may be a
result of intraplate plumes (e.g.
Hawaii), retrogradation of a subducting
slab, migration of back-arc spreading,
along-strike propagation of a rift (e.g.
East Africa), or propagation of a transform
fault with a transtensive component (the
Chagos ridge? see also Deccan
page). All these volcanic tracks may have
different depths of their mantle sources
and they must be differentiated (Figure
1). Plate boundaries by definition move
relative to one another and relative to
the underlying mantle. Therefore any hotspot
located on a plate boundary cannot be
used for a fixed-hotspot reference frame.
Figure
1. The main volcanic chains at the Earth's
surface may have different origins and
depths. The red arrows indicate the direction
of migration of volcanism with time. Filled
triangles represent the youngest volcanic
products. Volcanic trails originating
on ridges may be wetspots (in sensu Bonatti,
1990) and sourced from a fluid-rich asthenosphere.
The hotspots located on plate boundaries
are not fixed by definition, since both
ridges and trenches move relative to one
another and with respect to the mantle.
Pacific hotspots, regardless their source
depth, are located within the plate and
are virtually the only ones that can be
considered reliable for a hotspot reference
frame.
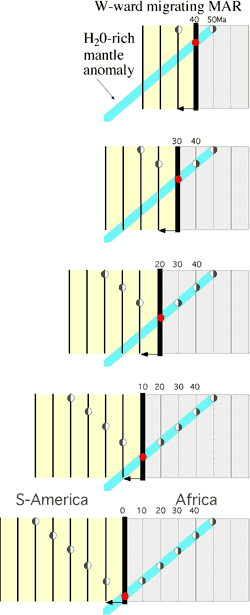 |
Deep
Plumes or Shallow Upwellings?
Fixed or Unfixed?
Accumulating evidence suggests
that hotspots are mostly shallow
features (Bonatti, 1900;
Smith & Lewis, 1999;
Anderson,
2000; Foulger,
2002; Foulger
et al.,
2005). For example, Atlantic
hotspots may be interpreted better
as wetspots (Figure 2) rather
than hot lines, as suggested by
Bonatti (1990). An asthenospheric
source richer in melting-point-lowering
fluids can account for magma overproduction.
Propagating rifts (hot-lines,
etc.) are shallow phenomena which
are not fixed relative to the
deeper mantle. The only hotspots
that are relevant to a fixed-hotspot
reference frame are those located
within plates. For example, Norton
(2000) grouped hotspots into three
main families, each of which has
very little internal relative
motion (the Pacific, Indo-Atlantic
and (single-hotspot) Iceland families).
He concluded that a global hotspot
reference frame is inadequate
because Pacific hotspots move
relative to Indo-Atlantic hotspots
and to Iceland. Since Indo-Atlantic
hotspots and Iceland are located
on ridges, they do not satisfy
the requirement for fixity. In
the analysis of Norton
(2000), Pacific hotspots have
been reasonably well fixed relative
to one another during the last
80 My. As a result, the screening
of volcanic tracks that may be
used for the fixed-hotspot reference
frame leaves a very limited number
of hotspots and only the Pacific
ones satisfy the requirements.
Decoupling
in the Asthenosphere
The origin of intraplate Pacific
magmatism is also obscure, and
the source depth and mechanism
of melting it is still under discussion
(Foulger
et al.,
2005). Since the Pacific is
the fastest-moving plate, shear
heating along the basal decollement
has been suggested as a potential
mechanism for generating localized
hotspots tracks (Figure 3). Areas
with viscosity higher than normal
in the asthenospheric decollement
should generate greater shear
heating.
Figure 2 Hypothetical reconstruction
of South Atlantic type migrating
volcanic ridges. An anomalously
water-rich asthenospheric mantle,
or wetline, oblique to absolute
African plate motion and Mid-Atlantic
Ridge (MAR) migration could generate
a SW-ward rejuvenating volcanic
track. Similar, mirror-image-like
tracks (NW-trending, SE-propagating)
could form in the South American
plate. This model could explain
why age progressive volcanic trails
are oblique to transform faults.
|
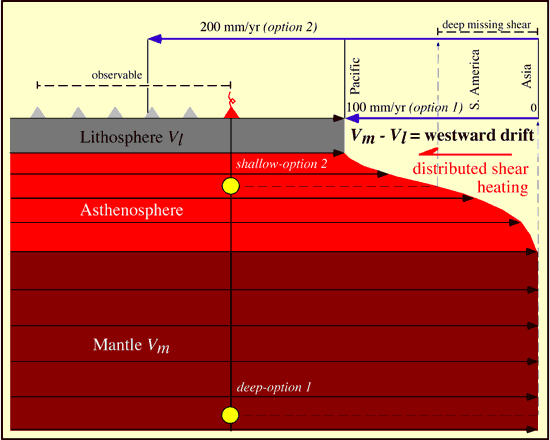
Figure
3 The Hawaiian volcanic track indicates
that there is decoupling between the magma
source and the lithosphere, which is moving
relatively toward the WNW. If the source
is below the asthenosphere (e.g., in the
sub-asthenospheric mantle, option 1),
the track records the entire shear between
lithosphere and mantle. In the case of
an asthenospheric source for the Hawaiian
hotspot (option 2), the volcanic track
does not record the entire shear between
the lithosphere and sub-asthenospheric
mantle, since part of it operates below
the source (deep missing shear). Moreover
the larger decoupling implies larger shear
heating, which could be responsible for
the scattered, punctiform Pacific intraplate
magmatism (after Doglioni
et al., 2005). [see also Hawaii
and Mantle Temperature
pages.]
Kennedy et al.
(2002) showed how mantle xenoliths record
shearing possibly located at the lithosphere-asthenosphere
interface. This supports the notion of
flow in the upper mantle and decoupling
at the base of the lithosphere, which
is also supported by seismic anisotropy
(Russo & Silver, 1996; Doglioni
et al.,
1999; Bokelmann & Silver,
2000). The fastest plate on Earth in the
hotspot reference frame (i.e.,
the Pacific) is also the one affected
by the most widespread intraplate magmatism.
It is noteworthy that the fast Pacific
plate overlies the asthenosphere with
the lowest mean viscosity (5 x 1017
Pa s; Pollitz et al., 1998),
and possibly the least depleted mantle,
and therefore the most prone to melting.
Because of the melting characteristics
of peridotite with minor carbon + hydrogen
(the lherzolite–(C+H+O) system),
the asthenosphere is already partially
molten (e.g., Schubert et al.,
2001) and at a temperature of about 1430°C
(e.g., Green & Falloon, 1998;
Green et al., 2001; see also
Mantle Temperature
page). A rise in temperature of only
a few tens of degrees will increase the
degree of melting and this melt, in a
deforming material, will migrate toward
the surface. We postulate that locally,
the viscosity of the asthenosphere can
also increase (e.g., up to 1019
Pa s) due to compositional anisotropy.
Shear stress could be irregularly distributed
in such inhomogeneous material, and consequently
higher shear heating (Shaw, 1973)
may develop locally and generate punctiform
magmatism (Figure 4).
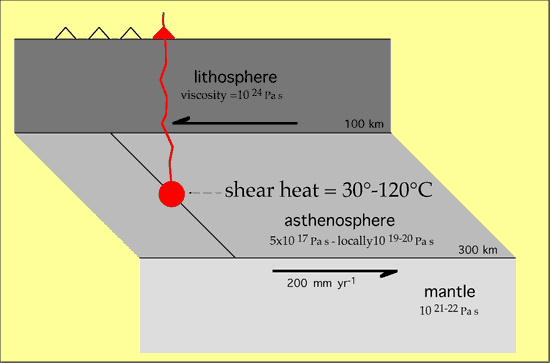
Figure 4. If locally
the viscosity of the asthenosphere is
higher than normal, the shear stress and
shear heating are also higher, providing
an increase in asthenospheric temperature.
Variations in lithosphere velocity (100
or 200 mm yr-1) and local increases
of the viscosity (4 x 1019
or 1020 Pa s) can determine
different excess temperatures ranging
between 12°K and 120°K. The highest
excess temperatures could result in extra
melting and possibly uprising intraplate
magmatism (after Doglioni
et al., 2005).
The
No-Net-Rotation Reference Frame
The NUVEL 1 model (DeMets
et al., 1990) and the Space Geodesy
ITRF2000 and NASA databases (Heflin
et al., 2005) provide information
on plate motions. They are based on the
artificially imposed assumption of a no-net-rotation
(NNR) reference frame, i.e.,
plates are imagined to move relative to
a fixed Earth center, and the lithosphere
does not move relative to the underlying
mantle, or the sum of its movements is
zero. However we know the lithosphere
does move relative to the sub-asthenospheric
mantle, and this is not only suggested
by the Hawaiian and similar volcanic tracks,
but it is also kinematically required
by the relative migration of plate margins.
The ITRF2000 reference frame (e.g.,
Heflin et al., 2005) is excellent
for describing relative plate motions,
and it is also considered to be an absolute
reference frame (relative to the GPS satellite
constellation and the Earth’s center
of mass). However, when magmatic sources
are included in the kinematic analysis,
the movement of the lithosphere relative
to the mantle must be taken into account
in an "absolute" plate motions
analysis, and the NNR must be abandoned.
Westward
Drift of the Lithosphere
When plate motions are measured
in the hotspot reference frame, the lithosphere
shows a net “westward” drift
(Bostrom, 1971; O'Connell
et al., 1991; Ricard et al.,
1991). This “westward” drift
persists also when plate motions are computed
relative to Antarctica (Le Pichon,
1968; Knopoff & Leeds, 1972),
which lies on a plate with almost no subducting
slab and is thus often considered to be
probably stationary, or moving only slowly,
with respect to underlying mantle. However
most of the hotspots used are neither
fixed, nor do they represent a fixed reference
frame, because they are located on plate
margins such as moving ridges (e.g.,
Galapagos,
Easter Island, Iceland,
and Ascension), transform faults (e.g.,
Reunion) or continental rifts (e.g.,
Afar), all of which are features that
are moving relative to one another and
relative to the mantle. Nevertheless,
Gripp & Gordon (2002) computed
a net rotation of the lithosphere of 49
mm yr-1 (0.44 ± 0.11
deg Myr-1 about a pole of 56°S,
70°E). The WNW-motion of the Pacific
plate relative to the underlying mantle
is inferred from the Hawaiian and other
major intraplate hotspot tracks (e.g.,
Marquesas, Society, Pitcairn, Samoan
and MacDonald), which show an average
velocity of about 103-118 mm yr-1.
They also move along the same trend (290°-300°,
WNW) and therefore they are the only hotspots
that appear to be coherently fixed relative
to one another, representing an apparently
reliable reference frame for absolute
plate motions.
Providing that the velocity
of the Pacific lithosphere in the ESE
(110-120°) direction is lower than
that of the underlying sub-asthenospheric
mantle Vm (Vm > Vl), the relative
motion corresponding to the WNW delay
of the lithosphere is Vm - Vl = 103
mm yr-1.
However, if the shear is distributed
throughout the asthenospheric channel
(Figure 3), and the Hawaiian melting
spot is within the asthenosphere (Figure
3, option 2) instead of the lower
mantle (Figure 3, option 1) then since
only the shear above the hotspot is
recorded in the hotspot trace, the
total displacement between the lithosphere
and mesosphere will be greater than
suggested by the surface volcanic
chain. Therefore if the location of
Hawaii magmatism is within or in the
upper part of the asthenosphere, there
should be a further deep missing component
of the shear, which would increase
the total relative velocity. This
higher velocity has two basic consequences,
1) it increases the global westward
drift of the lithosphere, and 2) it
increases the shear heating released
within the asthenosphere. If the source
of Pacific hotspots is located in
the middle of the asthenosphere, half
of the lithosphere-sub-asthenospheric
mantle relative motion is unrecorded,
which means that the total relative
displacement
would amount about 200 mm yr-1.
With this velocity, the net rotation
of the lithosphere toward the "west",
increases to about 9 cm/yr. In this
reference frame, no one plate moves "eastward"
relative to the mantle (Figure 5, option
2).
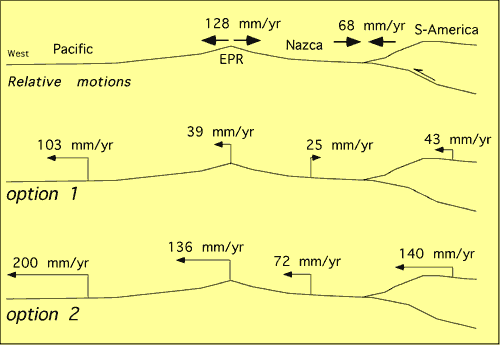
Figure 5. Simplified
kinematic relationship of the Pacific–Nazca–South
America plates. Relative motions vectors
(above) after Heflin et al. (2005). Option
1 indicates the “absolute”
motions relative to the Hawaiian hotspot
moving at about 103 mm yr-1
(Gripp & Gordon, 2002). Option 2 (below)
is the case where the hotspot source is
located in the asthenosphere and the relative
motion between Pacific plate and sub-asthenospheric
mantle is assumed to be ≥ 200 mm
yr-1 (see Figure 1). In this
last configuration all three plates move
“westward” relative to the
mantle.
Crazy
Tracks
There is evidence that the
propagation rate of Pacific “hotspots”
or seamount tracks has varied with time,
even with jumps back and forth and oblique
propagation relative to the “absolute”
plate motion, casting doubts on both the
notion of absolute plate motion computed
in the hotspot reference frame, and the
nature of the magmatism itself (deep plume,
or rather shallow upwellings generated
by cracks or boudins of the lithosphere,
Winterer & Sandwell, 1987;
Sandwell et al., 1995; Lynch,
1999; Natland & Winterer,
2003), sourced by a mantle with compositional
heterogeneity and no demonstrable thermal
anomaly in hotspot magmatism relative
to normal mid-oceanic ridges (see
also Mantle
Temperature page). Janney
et al. (2000) described the velocity
of the Pukapuka
volcanic ridge (interpreted as either
a hotspot track or a leaky fracture zone),
and located in the eastern Central Pacific,
between 5 and 12 Ma of about 200-300 mm
yr-1. They also inferred a
shallow mantle source for Pacific hotspots
based on their geochemical characteristics.
Relative plate
motions can now be estimated with great
accuracy using space geodesy (e.g.,
Robbins et al., 1993; Heflin et
al., 2005), refining the earlier NUVEL
1 plate motions model (DeMets et al.,
1990). The East Pacific Rise (EPR), separating
the Pacific and the Nazca plates, spreads
at rates of 128 mm yr-1 just
to the south of the equator (e.g., Heflin
et al., 2005). At the same latitude
the shortening along the Andean subduction
zone, where the Nazca plate subducts underneath
South America, has been computed to be about
68 mm yr-1. These relative motions,
when input into a reference frame where
the Hawaiian hotspot is considered fixed
and positioned in the sub-asthenospheric
mantle, imply that the Nazca plate moves
eastward relative to the sub-asthenospheric
mantle at about 25 mm yr-1 (Figure
5, option 1). If we assume that the source
of Pacific intraplate hotspots is rather
in the middle asthenosphere and half of
the lithosphere – sub-asthenospheric
mantle relative motion is missing in the
Hawaiian track (Figure 3), this motion could
increase to 200 mm yr-1, as also
suggested by some segments of the Pukapuka
volcanic ridge (Janney et al.,
2000). Note that in this configuration Nazca
would instead move west relative to the
mantle at 72 mm yr-1 (Figure 5, option 2)
and therefore all three plates would move
“westward” relative to sub-asthenospheric
mantle.
Shallow
Hawaii Upwellling
Another effect
of hypothesising a shallow source for Hawaiian
magmatism is to increase estimates of the
westward motion of the Pacific plate to
a velocity that is faster than the spreading
rate of the EPR, where the Nazca plate also
moves westward relative to the sub-asthenospheric
mantle (Figure 5, option 2). A shallow,
intra-asthenospheric origin of Pacific hotspots
provides a kinematic frame in which all
mid-oceanic ridges move “westward”.
As a consequence, ridges migrate continuously
over fertile mantle (Figure 6). They generate
melting and the increase in viscosity of
the residual mantle provides a mechanism
for slowing the plate motion.
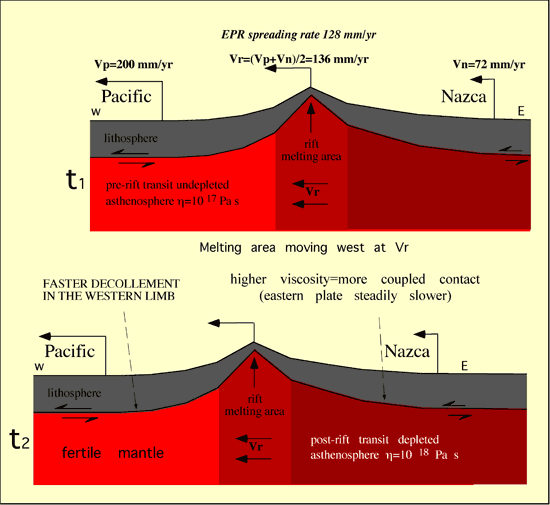
Figure
6. Assuming a fixed mantle, the Pacific
lithosphere moves “west” faster
than the Nazca plate because the underlying
asthenosphere is less viscous and the decoupling
is more efficient. Due to the increase in
viscosity and decrease in temperature along
the rifting area, which is also moving westward,
the asthenosphere below the eastern plate
is more viscous, causing stronger coupling
and a steady state lower velocity of the
Nazca plate. These kinematics provide continuous
new fertile mantle underneath the oceanic
ridge (after Doglioni
et al., 2005).
The deep and
shallow hotspot interpretations generate
two hotspot reference frames. In the case
of a deep mantle source for the hotspots,
a few plates still move “eastward”
relative to the mantle (Figure 7), whereas
in the case of shallow sources, all plates
have a “westward” component,
although with different velocities (Figure
8).
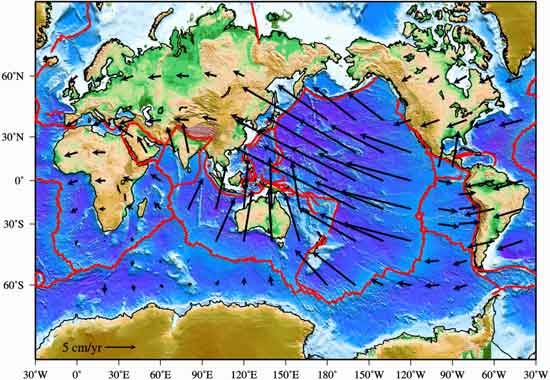
Figure
7. Current velocities with respect to the
deep-hotspot reference frame, option 1 of
Figure 3. Data from HS3-NUVEL1A (Gripp &
Gordon, 2002).
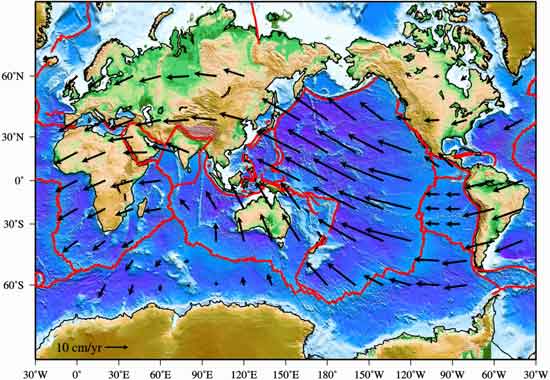
Figure
8. Present-day plate velocities relative
to the shallow-hotspot reference frame,
option 2 of Figure 3, incorporating the
NUVEL1A relative plate motions model. Note
that in this frame all plates have a westward
component.
These results are in accord with anisotropy
measured using shear-wave splitting (Russo
& Silver, 1994) and the low dip
of the Andean slab, which both suggest
relative eastward mantle flow. Similar
eastward mantle flow was proposed for
the North America plate (Silver &
Holt, 2002). The low dip of the Andean
slab has also been attributed to the age
of the subducting lithosphere. However
the oceanic age has been shown to be insufficient
to explain the asymmetry between “westerly”-directed
(steep and deep) vs. “easterly”-directed
(low dip and shallow) subduction zones
(Cruciani
et al.,
2005). In fact the geographically
related asymmetry persists even where
the same lithosphere (regardless whether
it is oceanic or continental) subducts
in both sides, such as in the Mediterranean
orogens (Doglioni
et al., 1999).
Global
Tectonic Asymmetries – Earth’s
Rotation?
Geological and geophysical signatures
of subduction and rift zones show a global
pattern, suggesting “eastward”
motion of the mantle relative to the lithosphere,
regardless the hotspot reference frame,
in support of a shallow origin for hotspots.
Plates move in a sinusoidal patern (Figure
9), which is largely confirmed by space-geodesy-derived
plate kinematics (e.g., Heflin et
al., 2005). Along the flow lines,
westerly dipping subduction zones are
steeper than those that are E- or NE-directed,
and associated orogens are characterized
by lower structural and topographic elevation,
and backarc basins, or by higher structural
and morphological elevation and no backarc
basins, respectively (Doglioni
et al.,
1999). The asymmetry is striking when
western and eastern Pacific subduction
zones are compared, and has usually been
interpreted as related to the age of the
downgoing oceanic lithosphere, i.e.,
older, cooler and denser in the west.
However these differences persist elsewhere,
regardless the age and composition of
the downgoing lithosphere, e.g.,
in the Mediterranean Apennines and Carpathians
vs. the Alps and Dinarides, or in the
Banda and Sandwich arcs, where even continental
or zero-age oceanic lithosphere is orientated
almost vertical along westerly directed
subduction zones. Rift zones are also
asymmetric, with the eastern flanks greater
elevated by about 100-300 m worldwide
(Doglioni
et al.,
2003).
Westward drift of the lithosphere implies
that plates have a general sense of motion
and are not moving randomly. If we accept
this postulate, we conclude that plates
move at different velocities toward the
"west" relative to the mantle
along the flow lines shown in Figure 9.
In this view, plates are more or less
detached with respect to the mantle as
a result of the decoupling at their base.
The degree of decoupling is mainly controlled
by the thickness and viscosity of the
asthenosphere. Lateral variations in degree
of decoupling could control the variable
velocity of the overlying lithosphere
(Figure 10). When a plate moves faster
westward compared with an adjacent plate
to the east, the resulting plate margin
is extensional; when a plate moves faster
westward compared with an adjacent plate
to the west, their common margin will
be convergent (Figure 10).
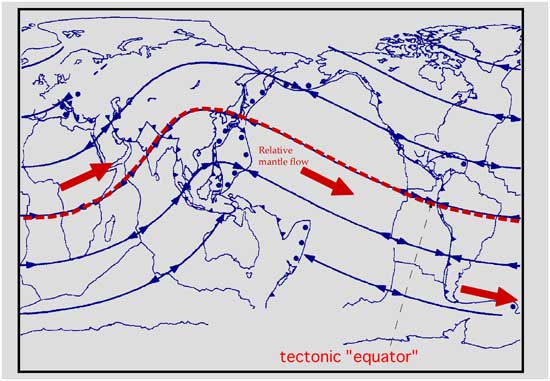
Figure 9. Connecting the directions
of absolute plate motions that we can
infer from large-scale rift zones or convergent
belts from the past 40 Ma, we observe
a coherent sinusoidal global flow field
along which plates appear to move at different
relative velocities in the geographic
coordinate system (after Doglioni,
1993).
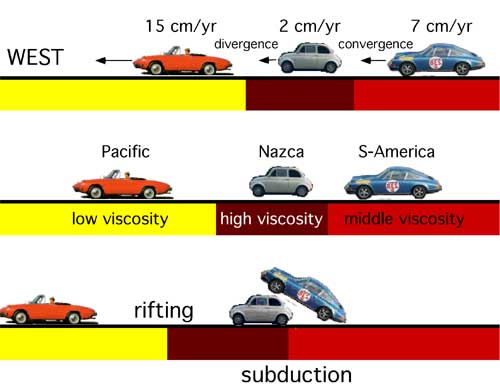
Figure 10. Cartoon illustrating that
plates (cars) move along a common trail
(e.g. the lines of Figure 9) but with
different velocities toward the west,
as indicated by the "westward"
drift of the lithosphere relative to the
mantle. The differential velocities control
the tectonic environment and result from
different viscosities in the decoupling
surface, i.e., the asthenosphere. There
is extension when the western plate moves
westward faster with respect to the plate
to the east, while convergence occurs
when the plate to the east moves westward
faster with respect to the plate to the
west. When the car in the middle is "subducted",
the tectonic regime switches to extension
because the car to the west moves faster,
e.g., the Basin & Range (after Doglioni,
1993).
The kinematic
framework of shallow Pacific hotspots
(Figure 8) constrains plate motions as
entirely polarized toward the west relative
to the deep mantle. This framework predicts
a fundamental observation along E- or
NE-directed subduction zones. In fact,
with this reference frame, slabs tend
to move out of the mantle, but are overridden
by the upper plates. This detracts from
slab pull as the mechanism for driving
plate motions since the slab then does
not sink into the mantle. In this view
slabs are rather passive features (Figure
11).
The global
scale asymmetry of tectonic features and
the westward drift of the lithosphere
supports a rotational component for the
origin of plate tectonics (Scoppola
et al.,
2006). The westward drift of the lithosphere
may be the result of three processes combined:
(1) tidal torques act on the lithosphere
generating a westerly directed torque
decelerating Earth’s spin; (2) downwelling
of denser material toward the bottom of
the mantle and in the core slightly decreases
the moment of inertia and speeds up Earth’s
rotation, only partly counterbalancing
tidal drag; and (3) thin (3–30 km)
layers of very low viscosity hydrate channels
occur in the asthenosphere. It is suggested
that shear heating and mechanical fatigue
self-perpetuate one or more channels of
this kind, which provide the necessary
decoupling zone of the lithosphere (Scoppola
et al., 2006).
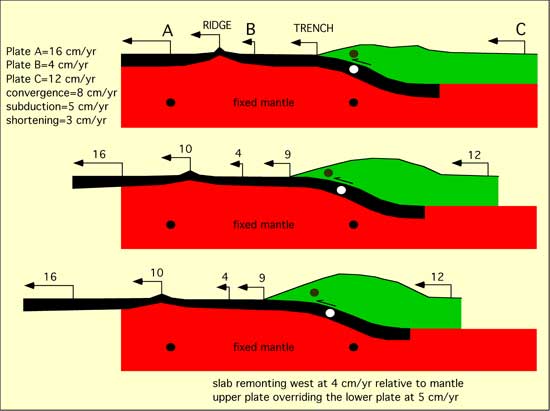
Figure
11. Cartoon assuming a Pacific plate (A)
moving at 16 cm/yr. When plate motions
are considered relative to the hotspot
reference frame, the slabs of E- or NE-directed
subduction zones may move out of the mantle.
This is clearly the case for Hellenic
subduction and, in the shallow hotspot
reference frame, also for Andean subduction.
This kinematic evidence of slabs moving
out of the mantle casts doubt on slab
pull as the driving mechanism of plate
motions.
-
-
Bokelmann
G.H., and Silver P.G. 2000.
Mantle variation within the
Canadian Shield: Travel times
from the portable broadband
Archean-Proterozoic transect
1989. J. Geophys. Res.,
105, 579–605.
-
Bonatti
E. 1990. Not So Hot "Hot
Spots" in the Oceanic Mantle,
Science, 250,
107-111.
-
Bostrom
R.C., 1971. Westward displacement
of the lithosphere, Nature,
234, 356-538.
-
-
DeMets
C., Gordon R.G., Argus F. and
Stein S. 1990. Current plate
motions. Geophys. J. Int.,
101, 425-478.
-
-
-
-
-
-
-
Green
D. H. and Falloon T.J., 1998.
Pyrolite: A Ringwood concept
and its current expression.
pp 311-380 in The Earth’s
Mantle; Composition, Structure,
and Evolution, ed I.N.S.
Jackson, Cambridge, Cambridge
University Press, 566 pp.
-
Green
D. H., Falloon T.J., Eggins
S.M. and Yaxley G.M. 2001. Primary
magmas and mantle temperatures.
European J. Min. 13,
437-451.
-
Gripp
A.E. and Gordon R.G. 2002. Young
tracks of hotspots and current
plate Velocities. Geophys.
J. Int. 150,
321-361.
-
-
Janney
P.E., Macdougall J.D., Natland
J.H. and Lynch M.A. 2000. Geochemical
evidence fom he Pukapuka volcanic
ridge system for a shallow enriched
mantle domain beneath the South
Pacific Superswell. Earth
Planet. Sci. Lett., 181,
47-60.
-
Kennedy
L.A., Russell, J.K., and Kopylova,
M.G. 2002. Mantle shear zones
revisited: The connection between
the cratons and mantle dynamics.
Geology, 30,
419-422.
-
Knopoff
L. and Leeds A. 1972. Lithospheric
Momenta and the Deceleration
of the Earth. Nature,
237, 93-95.
-
Le
Pichon, X., 1968, Sea-floor
spreading and continental drift,
J. Geophys. Res., 73,
3661-3697.
-
Lynch
M.A. 1999. Linear ridge groups:
evidence for tensional cracking
in the Pacific plate. J.
Geophys. Res., 104,
29,321-29,333.
-
-
Norton,
I.O., 2000. Global hotspot reference
frames and plate motion. In:
The history and dynamics
of global plate motions,
Richards, M.A., Gordon, R.G.
and Van der Hilst, R.D. (Eds),
Geophys. Monograph,
121, 339-357.
-
O'Connell,
R., Gable, C.G., and Hager,
B. 1991. Toroidal-poloidal partitioning
of lithospheric plate motions.
In: Glacial Isostasy, Sea-Level
and Mantle Rheology (Ed.
by R. Sabadini et al.), Kluwer
Academic Publisher, 334,
535-551.
-
Pollitz
F.F., Bürgmann R. and Romanowicz
B., 1998. Viscosity of oceanic
asthenosphere inferred from
remote triggering of earthquakes.
Science, 280,
1245-1249.
-
Ricard
Y., Doglioni C., and Sabadini
R. 1991. Differential rotation
between lithosphere and mantle:
a consequence of lateral viscosity
variations. J. Geophys.
Res., 96,
8407-8415.
-
Robbins
J.W., Smith D.E. and Ma C. 1993.
Horizontal crustal deformation
and large scale plate motions
inferred from space geodetic
techniques. In: Contributions
of Space Geodesy to Geodynamics:
Crustal Dynamics, Amer.
Geophys. Un., Geodynamics,
23, 21-36.
-
Russo,
R.M. and Silver, P.G. 1994.
Trench-parallel flow beneath
the Nazca plate from seismic
anisotropy. Science,
263, 1105-1111.
-
Russo,
R.M., and P.G. Silver, P.1996.
Cordillera formation, mantle
dynamics, and the Wilson cycle.
Geology, 24,
511–514.
-
Sandwell
D.T., Winterer E.L., Mammerickx
J., Duncan R.A., Lynch M.A.,
Levitt D.A. and Johnson C.L.
1995. Evidence for diffuse extension
of the Pacific plate from Pukapuka
ridges and cross-grain gravity
anomalies. J. Geophys. Res.,
100, 15,087-15,099.
-
-
Schubert
G., Turcotte D.L. and Olson
P., 2001. Mantle convection
in the Earth and Planets.
Cambridge University Press,
940 pp.
-
Shaw
H.R. 1973. Mantle convection
and volcanic periodicity in
the Pacific: evidence from Hawaii.
Geol. Soc. Amer. Bull.,
84, 1505-1526.
-
Silver
P.G. and Holt W.E. 2002. The
mantle flow field beneath western
north America. Science,
295, 1054-1057.
-
Smith,
A.D. and C. Lewis, 1999. The
planet beyond the plume hypothesis.
Earth Sci. Rev., 48,
135-182.
-
Winterer
E.L. and Sandwell D.T., 1987.
Evidence from en-echelon cross-grain
ridges for tensional cracks
in the Pacific plate. Nature,
329, 534-537.
|
last
updated 1st October, 2005 |