 |
|
Iceland
& the North Atlantic Igneous Province |
|
|
|
Click
here to go to News & Discussion of this page |
Abstract
It is almost universally assumed
that Iceland is underlain by a hot plume rising from
deep within the mantle. Nevertheless, this hypothesis
is inconsistent with many first-order observations at
Iceland, which is the best-studied ridge-centred hotspot
on Earth. There is essentially no evidence for very
high mantle temperatures, a time-progressive volcanic
track, a seismic anomaly in the lower mantle, or radial
symmetry in the pattern of geochemical anomalies on
land. Iceland is basically a melting anomaly underlain
by an upper-mantle seismic low-wave-speed anomaly. Temperatures
are only moderately, if at all, elevated above normal
mid-ocean ridge temperatures, the geochemistry is spatially
and temporally heterogeneous and it differs only subtly
from MORB.
Iceland lies where the mid-Atlantic
ridge crosses the Caledonian suture, which marks the
site of a ~ 400 Myr-old subduction zone. The great melt
production there may be explained by enhanced fertility
inherited from ancient subducted slabs that still remain
in the shallow mantle. This model is consistent with
the persistent locus of melt production on the ridge,
the lack of geophysical indicators of a plume and the
spatial and temporal geochemical heterogeneity of Icelandic
basalts.
In this way, Iceland and the associated
North Atlantic Igneous Province are explained as natural
consequences of relatively shallow processes related
to plate tectonics. This contrasts with the plume model,
where they are explained by a process entirely independent
of plate tectonics – a narrow diapiric upwelling
driven by heating at the surface of a deep, hot body
such as the Earth's core. |
Problems
with the plume model at Iceland
Few Earth science data from Iceland
and the North Atlantic Igneous Province agree with the
predictions of the plume hypothesis [Foulger,
2002]:
-
There is no evidence for the
250-600°C mantle temperature anomaly required
for a plume. Marine heat flow measurements around
Iceland provide no evidence for high temperatures
[ Stein
& Stein,
2003]. The geochemical compositional range of
Icelandic basalts overlaps that of normal mid-ocean
ridge basalt (N-MORB), and geothermometers yield
estimates of potential temperature (T p)
that range from temperatures similar to those inferred
to exist beneath mid-ocean ridges to being ~ 100
K higher. Even in central Iceland, primitive lavas
have eruptive temperatures of only ~ 1,240°C
[ Breddam, 2002], close to those of similarly
magnesian N-MORB [ Ford et al., 1983]. Picrite
glass, an indicator of high temperatures, is absent
from Iceland (see also Temperature
and Mantle
Temperature pages).
|
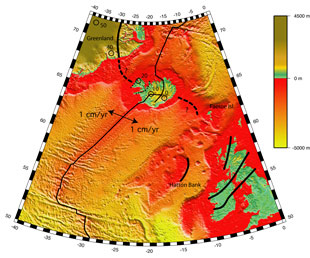
Figure 1: Bathymetry of
the north Atlantic. Thick lines indicate the Caledonian
suture. Circles indicate hypothesised locations of
an Icelandic mantle plume at the times indicated in
Ma.
|
-
For a plume at the latitude of
Iceland to have remained fixed with respect to other
Atlantic “hot spots”, it is required
to have migrated southeast from a location beneath
central Greenland at ~ 60 Ma to underlie southeast
Iceland at present [ Lawver & Muller,
1994]. However, no time-progressive volcanic track
such as occurs at Hawaii
is observed. Volcanism has been focused at the mid-Atlantic
ridge since the opening of the Atlantic at ~ 54
Ma (Figure 1; see also Iceland2
page). The proposed migration, which is widely assumed
and repeatedly asserted to have occurred, is unsupported
by data.
It is often asserted that the locus of spreading
within Iceland itself has migrated east since 15
Ma. However, the ages of surface lavas are more
consistent with a parallel pair of ridges having
existed in Iceland for much of the last 15 Myr,
and perhaps the last 26 Myr. Spreading in the approximate
location of the present-day Northern Volcanic Zone
(Figure 2: NVZ) has persisted, but spreading about
a subsidiary ridge in western Iceland has repeatedly
succumbed to transport off axis to the west, and
been replaced by new axes more colinear with the
Kolbeinsey and Reykjanes ridges (Figure 2). Simultaneous
spreading about more than one ridge is also supported
by geochemistry. Dated samples with ages of 7–2
Ma show that in eastern Iceland La/Sm and 87Sr/ 86Sr
decreased with age but in western Iceland they increased,
suggesting that the same mantle was not tapped in
the two areas [ Schilling et al., 1982].
The Iceland region classifies as a “diffuse
oceanic spreading plate boundary” [ Zatman
et al., 2001].
The resulting “leaky microplate” tectonics
gives rise to local complexities within Iceland
itself. These include variable rates and directions
of extension, which may explain the spatial and
temporal variations in magma production rate, and
the predicted existence of a captured oceanic microplate
with crust as old as ~ 30 Ma [ Foulger
& Anderson, 2005]. These complexities may
result from residual structure inherited from the
Caledonian suture, within which Iceland and the
Greenland-Iceland and Iceland-Faroe ridges formed.
The primary locus of spreading in Iceland, the Northern
Volcanic Zone (Figure 2: NVZ) has thus not migrated
east relative to the Kolbeinsey ridge as is often
claimed. It has, on the contrary, remained relatively
fixed since ~ 15 Ma and perhaps since ~ 26 Ma.
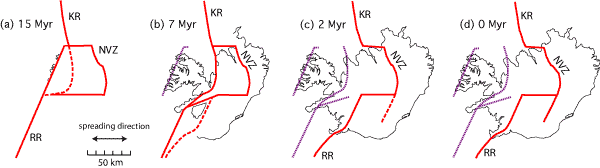
Figure 2: Tectonic evolution of Iceland during
the last 15 Myr. Red lines: active plate boundaries
(simplified), dashed red lines: imminent plate boundaries,
dashed mauve lines: extinct plate boundaries rafted
away in the westward-drifting North American plate,
KR, RR: Kolbeinsey and Reykjanes ridges, NVZ: Northern
Volcanic Zone [from Foulger,
2002].
-
Seismic tomography yields no
evidence for a plume-like structure in the lower
mantle. Iceland is underlain by a low-wave-speed
anomaly that all seismic tomography studies with
good upper-mantle resolution agree extends only
down to the mantle transition zone [ e.g., Ritsema
et al.,
1999; Figure 3].
Teleseismic tomography in Iceland itself reveals
that at depths greater than ~ 250 km the anomaly
becomes elongated in a direction parallel to the
mid-Atlantic ridge. This is consistent with an origin
that is not very much deeper [Figure 4; Foulger
et al., 2000; Foulger
et al.,
2001].
|
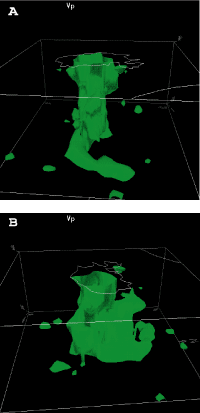
Figure 4: Three-dimensional
solid-body image of the delta-Vp > 0.3% low-wave-speed
anomaly beneath Iceland from the surface to 400
km depth. Map of Iceland on the surface provides
orientation: A) view from the north, B) view from
the east. From the model of Foulger
et al. [2000] and Foulger
et al.,
[2001]. |
|
Tomographic cross sections illustrating
a continuous, low-wave-speed body extending from the
surface to the core-mantle boundary beneath Iceland
have been produced [Bijwaard & Spakman,
1999] by:
-
Over-saturating the colour
scale, which imparts the visual impression of
continuity of strong anomalies in the upper mantle
and weak anomalies in the lower mantle. The latter
may be at the noise level and not confirmed by
other studies (e.g., compare Figures
3 & 5).
-
Cross sections are truncated
to remove similar bodies beneath the Canadian
shield and Scandinavia, where plumes are not expected
[Bijwaard & Spakman, 1999].
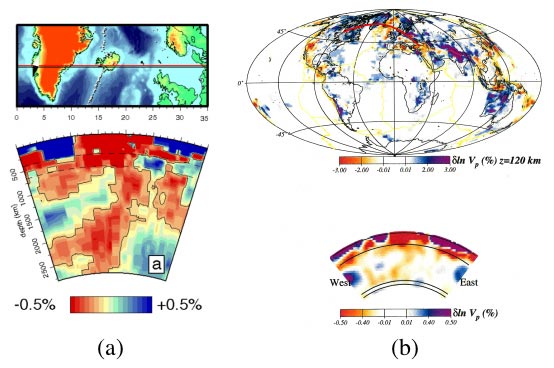
Figure 5: (a) Cross section showing a low-wave-speed
anomaly beneath Iceland that is apparently continuous
from the surface to the core-mantle boundary (from
Bijwaard & Spakman, 1999). The colour scale is
saturated at an anomaly strength of Vp = 0.5%. (b)
the same line of section, but longer, through the
same model, plotted using a colour scale saturated
at a higher value. The anomaly beneath Iceland is
clearly much weaker in the lower mantle, and similar
lower-mantle anomalies are also observed beneath Hudson
Bay, Canada, and Scandinavia. The downward-continuous
anomaly beneath Iceland is not confirmed in other
studies e.g., that of Ritsema
et al. [1999]
(Figure 3).
|
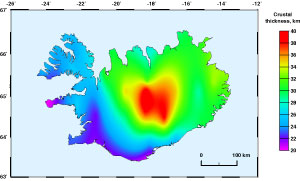
Figure 6a: Map showing the depth to the
base of the lower crust (defined as the depth
to the Vs = 4.2 km/s horizon), from receiver
functions [from Foulger
et al., 2003]. Click on figure for enlargement.
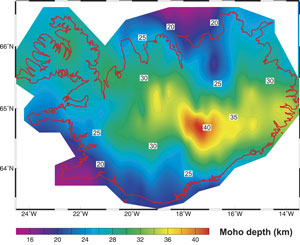
Figure 6b: Map of total crustal thickness
determined using a combination of explosion
seismology profiles and gravity [from Darbyshire
et al., 2000]. Click on figure for enlargement.
|
-
Crustal structure in
Iceland is not what is predicted by the
plume hypothesis. Maps of crustal thickness
show that the crust is thickest, ~ 40 km,
beneath central Iceland and thins to 20-30
km toward the coasts (Figure 6). This is
not what is expected for a plume over which
the lithosphere is moving. The crust is
thinner beneath western Iceland than eastern
Iceland, the opposite of what is expected
for an eastward-migrating plume. The local
gravity field can probably be explained
by crustal structure alone, without the
need for density anomalies in the mantle.
The block of thick crust beneath central
Iceland may be a submerged, captured oceanic
microplate (compare Figures 2 & 6a)
[ Foulger
& Anderson, 2005].
Historically, crustal seismic data from
Iceland have been interpreted both as indicating
that the crust is thin and the mantle beneath
hot (the “thin, hot” model),
and that the crust is thick and the mantle
beneath cool (the “thick, cold”
model). Both of these models have been considered
to be consistent with the plume hypothesis,
illustrating well that the model of a plume
beneath Iceland is a data-independent a-priori
assumption, and not an hypothesis [ Foulger
et al.,
2003]. Current interpretations of crustal
seismic data suggest that the crust beneath
Iceland is cooler than at similar depths
beneath the East-Pacific Rise [ Menke
& Levin, 1994].
|
-
There is no evidence in seismic
anisotropy for the pattern of radial flow expected
from a plume beneath central or south-central Iceland
[Li & Detrick, 2003] (Figures 7 &
8). The results are, instead, consistent with flow
parallel to the rift zones in Iceland at depths
< 100 km, and in a general NS direction or parallel
to the mid-Atlantic ridge at depths > 100 km
[Li & Detrick, 2003].
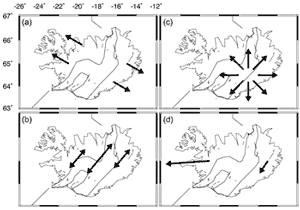
Figure 7: Possible mantle
flow components beneath Iceland, (a) in plate
spreading direction. (b) channeled along the ridge,
(c) Radial from a plume centre, (d) from absolute
plate movement [from Li & Detrick, 2003]. |
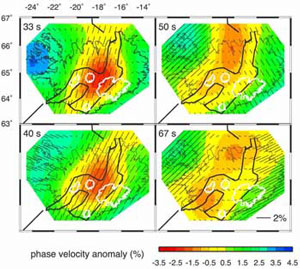
Figure 8: Maps of Rayleigh-wave
phase velocity and azimuthal anisotropy at four
periods (33, 40, 50 and 67 s). Colour contours
are isotropic phase velocity perturbations relative
to the average values for Iceland. As a rule of
thumb, for these depths and periods, the depths
sensed in km are approximately equal to the period
in seconds (given at top left in each panel).
Strikes of lines show orientations of fast directions,
which may indicate flow direction. Line
lengths are proportional to the magnitude of anisotropy
[from Li & Detrick, 2003]. |
-
There is no geochemical evidence
for a localised magma source beneath SE-central
Iceland (Figure 1) or radial flow from this location:
-
The geochemistry of Icelandic
rocks cannot be explained simply by mixing
of an “enriched” plume component
and a “depleted” MORB component,
but additional components must be invoked,
e.g., to explain the helium isotope
ratios (see also Helium
Fundamentals page). It has been proposed
that as many as five components are required
to explain the geochemistry, and that the
“Icelandic plume” is heterogeneous.
The spatial pattern of heterogeneities does
not reveal a radial pattern.
-
Pb isotope ratios do
not increase towards the proposed plume
location beneath SE-central Iceland (Figure
1). The lowest Pb isotopic compositions
are not restricted to samples located on
the edge of Iceland [ Chauvel
& Hemond,
2000], and some highly unradiogenic
Pb values are found in central Iceland.
Furthermore, Pb isotope values correlate
with rock type and not location.
- Immediately north of the proposed plume centre,
Pb is relatively unradiogenic (206Pb/204Pb,
207Pb/204Pb and 208Pb/204Pb
are low) in the Northern Volcanic Zone and along
the Kolbeinsey ridge, suggesting depleted mantle
sources for each [Mertz et al., 1991].
87Sr/86Sr, also postulated
to be a plume tracer, decreases towards
SE-central Iceland from the Icelandic shelf
edge [Schilling et al., 1983], the
opposite of what is expected. 3He/4He
is lower in the Northern Volcanic Zone than
on the more distant and indirectly linked Reykjanes
peninsula. La/Sm ratios, also expected to increase
towards a plume centre, decrease southward
along the Kolbeinsey ridge whereas they increase
northward along the Reykjanes ridge towards
Iceland [Mertz et al., 1991]. There
is thus no geochemical evidence for northward
flow of “plume” material from Iceland
along the Kolbeinsey ridge [Mertz et al.,
1991]. In addition to being inconsistent with
the plume model, this also casts doubt that
the poorly developed chevron (“V-shaped”)
ridges about the Kolbeinsey ridge formed by
the northward flow of pulses of hot “plume”
material from SE-central Iceland.
- There is a discontinuity in many chemical
species across central Iceland, e.g.,
La/Sm and 87Sr/86Sr between
the Western Volcanic Zone and the Skagi Zone
[Schilling et al., 1982].
-
Other geochemical problems associated
with the plume model at Iceland include:
-
87Sr/86Sr
and 143Nd/144Nd are not
correlated with 3He/4He
[Condomines et al., 1983].
-
The Kolbeinsey ridge, like
the Reykjanes ridge, is shallow. However, it
appears to bear little geochemical evidence
that it is supported by “plume”
material, which suggests that such an explanation
for the shallow bathymetry of the Reykjanes
ridge is not unique [Mertz et al.,
1991].
-
It is commonly assumed that
plumes are HIMU, i.e., they are enriched
in 238U/ 204Pb. However,
such rocks comprise only the alkaline basalts
in Iceland, that represent a small percentage
of all the lavas. The majority are tholeiites
that are low in 206Pb/ 204Pb
[ Chauvel
& Hemond,
2000].
-
Different “plume tracers”
do not agree, e.g., elevated 87Sr/86Sr
and delta-Nb extend only as far south as 61°N
[e.g., Fitton et al., 1997], but 3He/4He
is elevated as far south as the Charlie Gibbs
Fracture Zone at 53°N.
- Shorter crustal residence times, concluded
to be a response to glacial unloading, are observed
to make erupted lavas more depleted [Gee et
al., 1998], illustrating that crustal processes
influence lava characteristics that might otherwise
be interpreted in terms of a plume model.
|
What exactly
needs to be explained at Iceland?
In order to explain Iceland and the
associated volcanic province, a model is required that
can account for:
- the production of 2-3 times more melt at the
mid-Atlantic ridge between ~ 63°30' and ~ 66°30'
than elsewhere, at temperatures at most relatively
mildly elevated,
- a regional mantle seismic anomaly that extends
down to the transition zone, in contrast with the
~ 250-km depth extent of low-wave speeds beneath
marine parts of the spreading ridge system, and
- the ocean-island-basalt (OIB)-like geochemistry
of some of the lavas.
A shallow model involving
processes related to plate tectonics
In the absence of high temperatures,
mantle composition that is more fusible than normal
peridotite is probably the only option. Iceland and
the North Atlantic Volcanic Province formed in the Caledonian
suture, which was created at ~ 400 Ma when what is now
Greenland and Scandinavia collided as the Iapetus ocean
closed (Figure 9). In particular, the province formed
where the new mid-Atlantic ridge crossed the western
frontal thrust where it traverses the north Atlantic
from Greenland to Britain. Because the Caledonian suture
is the site of earlier subduction, abundant eclogite
is expected. Eclogite is the high-pressure form of basalt,
and is created when oceanic crust is carried deep into
the Earth at subduction zones.
Figure 9: Closure of the Iapetus
ocean at (a) 440 Ma, and (b) 400 Ma, by convergence
of Laurentia, Baltica and Avalonia. Arrows: convergence
directions; thick lines: faults and orogenic fronts.
Black triangles indicate the sense of thrust faults.
Slabs were subducted beneath Greenland, Baltica and
Britain. Dashed red line indicates the position of the
mid-Atlantic ridge that formed at ~ 54 Ma [from Foulger
& Anderson,2005]. |
|
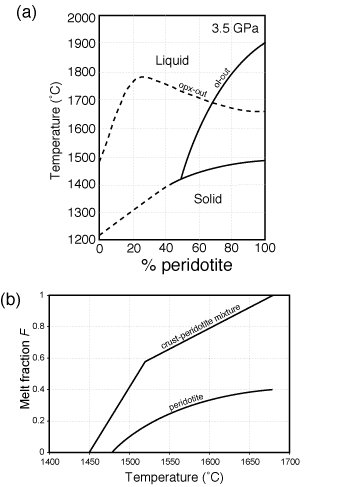
|
Eclogite, and eclogite-peridotite mixtures,
have lower liquidi and solidi and a narrower melting
interval than peridotite [Yaxley, 2000]
(Figure 10a). At typical mantle temperatures,
where peridotite melts to the extent of just a
few percent, eclogite may be almost completely
molten. In the case of eclogite-peridotite mixtures,
up to several times the amount of melt is expected
than from pure peridotite (Figure 10b). Thus it
may be possible to explain the volume of melt
at Iceland by processes the same as elsewhere
along the mid-Atlantic ridge, but occurring where
the mantle is fertilised by eclogite in the ancient
subduction zone within which it lies. This model
suggests that Iceland can be explained by passive
upwelling of unusually fertile mantle. It predicts
that that isentropic decompression melting of
an eclogite-rich source at the mildly elevated
temperatures of the north Atlantic can produce
2 – 3 times as much melt volume as the same
process involving peridotite only. |
Figure 10:
(a) Solidus and liquidus for fertile peridotite
containing varying percentages of average altered
oceanic crust. opx: orthopyroxene, ol: olivine
[adapted from Yaxley, 2000], (b) Relationship
between melt fraction F and temperature for fertile
peridotite and a mixture of 30% average altered
oceanic crust and 70% fertile peridotite. The
peridotite line is the parameterisation of McKenzie
& Bickle [1988] for normal fertile peridotite,
and the crust-peridotite line is an approximate
estimate for the bulk composition corresponding
to the liquidus minimum of (a). The higher average
dF/dT and lower solidus temperature for the mixture
results in enhanced melt productivity at a given
temperature [derived from data in Yaxley, 2000]. |
|
There is plentiful geochemical
evidence for remelted crust of Caledonian age
in the basalts of east Greenland, Iceland and
Britain from calculated compositions of parental
melts, trace- and rare-earth elements and radiogenic
isotope ratios [Breddam, 2002; Chauvel
& Hemond,
2000; Fitton et al., 1997; Korenaga
& Kelemen,
2000; Lesher et al., 2002].
Na8 and TiO2
are much higher in Iceland than on the adjoining
ridges, the opposite of what is expected [Klein
& Langmuir, 1987] for the greater extent
of melting and depth range of melting required
to explain the thicker crust, assuming a peridotite
source (Figure 11). A peridotite/eclogite mixture
is expected to have high Na8 and TiO2.
A source in extensively melted
subducted Iapetus crust can explain the subtle
differences in geochemistry between Icelandic
basalts and MORB, including rare-earth elements,
trace element ratios such as Zr/La, Nb-Y-Zr systematics,
isotopic and noble-gas data [Foulger
et al., 2005].
Oceanic crust comprises a variety of lithologies,
including troctolite, olivine gabbro, gabbronorite,
oxide gabbro and minor residual granitic material.
Remelting these produces basalts that reflect
subtle variations in geochemistry inherited from
the fractionation history of the corresponding
mineralogy.
|
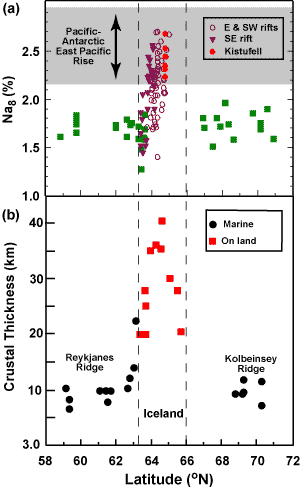 |
Figure 11:
(a) Parental soda (Na8) in basalt glass
v. latitude. (b) Crustal thickness vs. latitude,
from a compilation of seismic experiments in Iceland
and the North Atlantic [from Foulger
et al., 2003]. |
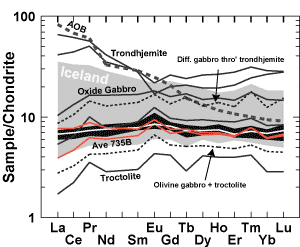
Figure 12: Comparison of the
rare-earth-element patterns of average Hole 735B
gabbro lithologies with and without the addition
of alkali olivine basalt (AOB), with those of
Icelandic basalts [from Foulger
et al.,2005].
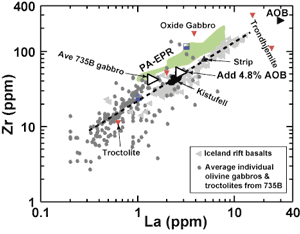
Figure 13: Icelandic basalts
can be well modelled as average gabbro from DSDP
hole 735B plus 4.8% alkali olivine basalt [Foulger
et al.,2005].
|
What has been termed the “depleted plume
component” in Icelandic basalts may be
derived from remelted abyssal olivine gabbro
[Chauvel
& Hemond,
2000; Kempton et al., 2000; Breddam,
2002]. Icelandic ferrobasalts are similar to
abyssal oxide gabbros. The “enriched plume
component” may be derived from remelting
axial or seamount E-MORB, alkalic olivine basalt,
associated intrusive rocks and sedimentary materials
of the subducted crust, or possibly small amounts
of ancient continental crust that may, as at
Jan Mayen, still underlie portions of Iceland
[Foulger
et al., 2005; Amundsen et al.,
2002].
ODP Hole 735B cored a very long section of
gabbroic oceanic crust on the southwest Indian
ridge, and may be used to estimate the composition
of subducted oceanic crust. The chondrite-normalized
rare-earth-element patterns of primitive basalts
from Kistufell in central Iceland [Breddam,
2002] may be modelled using the Hole 735B data.
In Figure 12, average Hole 735B gabbro lithologies
(solid black lines) are compared with the range
(black band) and average (white line) of basalts
from Kistufell [Breddam, 2002]. The
range of basalts from Icelandic rifts [MacLennan
et al., 2002] is shown as the gray field.
The bold dashed line shows the average of two
Ne-normative alkali-olivine basalts (AOB) from
seamounts near the East Pacific rise. The two
red lines show the weighted average of all samples
analyzed for rare-earth elements from Hole 735B
(lower red line), and the same composition plus
4.8% AOB (upper red line). The latter closely
matches average Kistufell olivine tholeiite.
In general, the flat-to-enriched REE patterns
of Icelandic basalts can be produced by mixing
melt derived from abyssal gabbro and either
an enriched material such as AOB, or silicic
material, such as trondhjemite.
|
Figure 13 illustrates
how Zr and La can be modelled in a similar way. |
The high 3He/ 4He observed in Iceland
is probably of Caledonian age, and preserved in olivine
crystals in the subducted slabs. Olivine traps helium
in gas bubbles, and since U+Th is essentially absent from
olivine crystals, old, high 3He/ 4He
ratios are preserved until such time as the olivine is
remelted (see Helium
fundamentals page) [ Anderson, 1998; Natland,
2003]. |
Physical models for
melting subducted crust
The extent to which subducted
crust trapped at shallow levels in the mantle
re-homogenizes with its peridotite host is not
known. The retention of essentially pristine blocks
of crust that are of the order of kilometres in
thickness, and complete homogenization with mantle
peridotite, represent end-member scenarios. For
the case of extensive melting of pristine blocks
of crust, the experiments of Ito & Kennedy
[1974] and the compositions of natural gabbros
suggest that 60-80% melting of the original bulk
gabbroic assemblage is required to reproduce the
compositions of Icelandic tholeiites.
Eclogite upwelling beneath the
mid-Atlantic ridge in the Iceland region will
be much closer to its solidus than peridotite,
or it may even be partially molten. Experimental
work shows that the crystallization/melting interval
for eclogite (80 – 90 K) is smaller than
for gabbro (> 200 K), and much smaller than
for peridotite (~ 400 K) [Yoder & Tilley,
1962; Ito & Kennedy, 1974]. A melt
equivalent to a moderately differentiated abyssal
tholeiite can thus probably be generated from
an average eclogitic abyssal gabbro, and partial
melting beyond a few tens of percent will not
greatly affect the generally basaltic composition. |
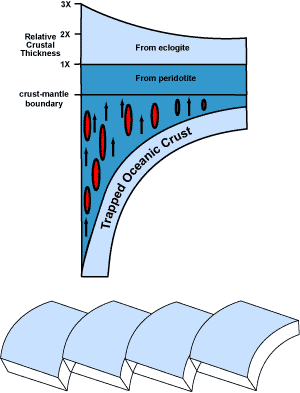
Figure 14: Schematic diagram illustrating
how anomalously large amounts of melt may be
obtained from remelting a subducted crustal
slab of normal thickness. The slab may be emplaced
at a high angle in the mantle (top) or thickened
by imbrication (bottom).
|
Melt extraction from partially molten
rock can begin at degrees of melting of less than one
percent, and consequently, progressively extracted melt
increments derived from eclogite must pond and re-homogenize
in some reservoir prior to eruption. A similar process
of fractional melting, aggregation, and homogenization
is also required beneath normal spreading ridges since
MORB is thought to be formed by up to ~ 20% partial
melting of peridotite integrated over the melt column.
In order to consistently produce 2
– 3 times the normal thickness of crust in the
north Atlantic (~ 10 km), more than one “normal
thickness” of subducted oceanic crust is required.
This could be available if the recycled crustal slabs
are emplaced at a steep angle in the mantle, or imbricated
(Figure 14). In the case of eclogite dispersed in a
host of peridotite, the amount of fusible material available
would depend on the degree of refertilisation that took
place and the depth extent of the melt source region. |
Plate
tectonics beginning and end games
The opening and closing stages of oceans
and the formation of collisional sutures are probably
radically different processes from steady-state plate
tectonics. The final stages of ridge-trench collision
introduces sediments, water, back-arc basins and young,
thin, hot oceanic crust into the shallow mantle. Such
lithosphere is buoyant, and thermal modelling suggests
that if younger than ~ 50 Myr, it does not sink deeper
than a few hundred km [Oxburgh & Parmentier,
1977]. At a half-spreading rate of 1 cm/a, this would
amount to 500 km of plate. A length of the final subducting
lithosphere equivalent to the thickness of the colliding
cratons, or up to ~ 200 km would be trapped in the continental
lithosphere. The remainder of the late-subducting, buoyant
oceanic lithosphere, perhaps up to several hundred km
in length, might be retained in the asthenosphere beneath
the sutured cratons.
When continental break-up occurs along
old sutures, magmatism may be enhanced by mantle made
unusually fertile by eclogitised subducted oceanic crust
trapped in the rifting lithosphere, and this may contribute
to the formation of volcanic margins. Delamination of
the continental mantle lithosphere at the time of break-up
may also recycle fertile material into the mantle beneath
the new ocean. Enhanced magmatism may continue longer
than the initial break-up stage if subducted material
lies in belts transverse to the new ridge, and continues
to be recycled into the melt extraction zone beneath
the ridge despite lateral ridge migration. That lateral
ridge migration with respect to underlying mantle occurs
is shown by the fact that globally ridges migrate with
respect to one another. A model involving a transverse
belt of fertility may also explain magmatism in the
Tristan da Cunha region in the south Atlantic, and elsewhere.
|
New
questions and problems
The model described above does not
suffer from many of the problems of the plume hypothesis,
but it nevertheless raises several new questions and
problems. Some of these are:
-
Can isentropic upwelling provide
enough thermal energy to produce the large melt
volumes observed from eclogite, or peridotite refertilised
with recycled eclogite?
-
Can large melt fractions, perhaps
up to 60–80%, be ponded and homogenised above
the melting column, and at what depth might this
occur?
-
Eurasia drifted through several
tens of degrees of latitude between 400 and 50 Ma.
Down to what depth did the upper mantle drift with
it?
- Why are large quantities of ecolgite not observed
in exhumed orogenic belts?
A model such as is described here,
which is radically different from the plume model, may
require us to rethink related assumptions about structure
and processes inside the Earth. |
Concluding remarks
A model whereby the Iceland melting
anomaly is derived from shallow sources in the mantle
and processes consequential to plate tectonics (see
also Anderson
[2001] and PT
Processes page) is consistent with the absence
of very high temperatures, the persistence of the
melting anomaly on the mid-Atlantic ridge, the seismic
tomography, crustal structure and geochemistry. It
is also consistent with the north-south tectonic and
geochemical asymmetry, which is expected if rifting
occurs above a heterogeneous mantle harbouring north-south
asymmetry inherited from an embedded dipping slab
that has variable melt fertility. Above such a heterogeneous
mantle, small structures, e.g., the Tjornes
Fracture Zone in north Iceland, are expected to be
marked by abrupt changes in geochemistry, and this
is indeed observed. In the model proposed here, the
location of the Iceland volcanic province over an
easterly trending branch of the Caledonian suture
is not a coincidence.
|
News
& Discussion
No
Plume Under Iceland
Iceland
plume: four articles, pro and con
|
References
-
Amundsen, H.E.F., U. Schaltegger,
B. Jamtveit, W.L. Griffin, Y.Y. Podladchikov, T.
Torsvik, and K. Gronvold, Reading the LIPs of Iceland
and Mauritius, in 15th Kongsberg Seminar,
edited by B. Jamtveit, and H.E.F. Amundsen, Kongsberg,
Norway, 2002.
-
Anderson, D.L., A model to explain
the various paradoxes associated with mantle noble
gas geochemistry, Proc. Nat. Acad. Sci.,
95, 9087-9092, 1998.
-
-
Bijwaard, H., and W. Spakman,
Tomographic evidence for a narrow whole mantle plume
below Iceland, Earth planet. Sci. Lett.,
166, 121-126, 1999.
-
Breddam, K., Kistufell: Primitive
melt from the Iceland mantle plume, J. Pet.,
43, 345-373, 2002.
-
-
Condomines, M., K. Gronvold,
P.J. Hooker, K. Muehlenbacks, R.K. O'Nions, N. Oskarsson,
and E.R. Oxburgh, Helium, oxygen, strontium and
neodymium isotopic relationships in Icelandic volcanics,
Earth planet. Sci. Lett., 66,
125-136, 1983.
-
Darbyshire, F.A., White, R.S.
& Priestley, K.F., Structure of the crust and
uppermost mantle of Iceland from a combined seismic
and gravity study, Earth planet. Sci. Lett.,
181, 409-428, 2000.
-
Fitton, J.G., A.D. Saunders,
M.J. Norry, B.S. Hardarson, and R.N. Taylor, Thermal
and chemical structure of the Iceland plume, Earth
planet. Sci. Lett., 153, 197-208,
1997.
-
Ford, C.E., D.G. Russell, J.A.
Craven, and M.R. Fisk, Olivine-liquid equilibria:
temperature, pressure and composition dependence
of the crystal/liquid cation partition coefficients
for Mg, Fe2+, Ca, and Mn, J. Pet.,
24, 256-265, 1983.
-
-
- Foulger,
G.R., Z.J. Du and B.R. Julian, Icelandic type crust
,Geophys. J. Int. ,155 ,
567-590, 2003.
-
- Foulger,
G.R., M.J. Pritchard, B.R. Julian, J.R. Evans, R.M.
Allen, G. Nolet, W.J. Morgan, B.H. Bergsson, P. Erlendsson,
S. Jakobsdottir, S. Ragnarsson, R. Stefansson, and
K. Vogfjord, Seismic tomography shows that upwelling
beneath Iceland is confined to the upper mantle, Geophys.
J. Int., 146, 504-530, 2001.
- Foulger,
G.R., M.J. Pritchard, B.R. Julian, J.R. Evans, R.M.
Allen, G. Nolet, W.J. Morgan, B.H. Bergsson, P. Erlendsson,
S. Jakobsdottir, S. Ragnarsson, R. Stefansson, and
K. Vogfjord, The seismic anomaly beneath Iceland extends
down to the mantle transition zone and no deeper,
Geophys. J. Int., 142, F1-F5,
2000.
-
Gee, M.A.M., R.N. Taylor, M.F.
Thirlwall, and B.J. Murton, Glacioisostacy controls
chemical and isotopic characteristics of tholeiites
from the Reykjanes peninsula, SW Iceland, Earth
planet. Sci. Lett., 164, 1-5,
1998.
-
Ito, K., and G.C. Kennedy, The
composition of liquids formed by partial melting
of eclogites at high temperatures and pressures,
J. Geol., 82, 383-392,
1974.
-
Kempton, P.D., J.G. Fitton, A.D.
Saunders, G.M. Nowell, R.N. Taylor, B.S. Hardarson,
and G. Pearson, The Iceland plume in space and time:
a Sr-Nd-Pb-Hf study of the north Atlantic rifted
margin, Earth planet. Sci. Lett., 177,
273-285, 2000.
-
Klein, E.M., and C.H. Langmuir,
Ocean ridge basalt chemistry, axial depth, crustal
thickness and temperature variations in the mantle,
J. geophys. Res., 92,
8089-8115, 1987.
-
-
Lawver, L.A., and R.D. Muller,
Iceland hotspot track, Geology, 22,
311-314, 1994.
-
Lesher, C.E., J. Blichert-Toft,
and O. Stecher, Mantle sources and contamination
of the basalts of the North Atlantic Igneous Province,
submitted to J. Pet., 2002.
-
Li, A.B., and R.S. Detrick, Azimuthal
anisotropy and phase velocity beneath Iceland: implication
for plume-ridge interaction, Earth planet. Sci.
Lett., 214, 153-165, 2003.
-
Maclennan, J., M. Jull, D. McKenzie,
L. Slater, and K. Gronvold, The link between volcanism
and deglaciation on Iceland, Geochem. Geophys.
Geosyst., 3, 2001GC000282,
1-25, 2002.
-
McKenzie, D., and J. Bickle,
The volume and composition of melt generated by
extension of the lithosphere, J. Pet.,
29, 625-679, 1988.
-
Menke, W., and V. Levin, Cold
crust in a hot spot, Geophys. Res. Lett.,
21, 1967-1970, 1994.
-
Mertz, D.F., C.W. Devey, W. Todt,
P. Stoffers, and A.W. Hofmann, Sr-Nd-Pb isotope
evidence against plume-asthenosphere mixing north
of Iceland, Earth planet. Sci. Lett., 107,
243-255, 1991.
-
Natland, J.H., Capture of mantle
helium by growing olivine phenocrysts in picritic
basalts from the Juan Fernandez Islands, SE Pacific,
J. Pet., 44, 421-456,
2003.
-
Oxburgh, E.R., and E.M. Parmentier,
Compositional and density stratification in oceanic
lithosphere - causes and consequences, J. geol.
Soc. Lon., 133, 343-355, 1977.
-
-
Schilling, J.-G., P.S. Meyer,
and R.H. Kingsley, Evolution of the Iceland Hotspot,
Nature, 296, 313-320,
1982.
-
Schilling, J.-G., M. Zajac, R.
Evans, T. Johnston, W. White, J.D. Devine, and R.
Kingsley, Petrologic and geochemical variations
along the mid-Atlantic ridge from 29ÚN to 73ÚN,
Am. J. Sci., 283, 510-586,
1983.
-
-
Yaxley, G.M., Experimental study
of the phase and melting relations of homogeneous
basalt + peridotite mixtures and implication for
the petrogenesis of flood basalts, Cont. Min.
Pet., 139, 326-338, 2000.
-
Yoder, H.S., Jr. and Tilley,
C.E., Origin of basalt magmas: an experimental study
of natural and synthetic rock systems. J. Pet.,
3, 342-532, 1962.
-
Zatman, S., R.G. Gordon, and
M.A. Richards, Analytic models for the dynamics
of diffuse oceanic plate boundaries, Geophys.
J. Int., 145, 145-156, 2001.
|
last
updated 8th February, 2005 |
|
|