 |
|
The
Emperor and Hawaiian Volcanic Chains: How
well do they fit the plume hypothesis?
|
Kilauea Lava, Hetu Sheth |
|
|
Click here for
News & Discussion of this page |
A
note on Pele
Pele was born of the female spirit
Haumea, or Hina, who, like all other important Hawai`i
gods and goddesses, descended from the supreme beings,
Papa, or Earth Mother, and Wakea, Sky Father. Pele was
among the first voyagers to sail to Hawai`i, pursued,
legends say, by her angry older sister, Na-maka-o-kaha`i
because Pele had seduced her husband. Pele landed first
on Kauai`i, but every time she thrust her o`o (digging
stick) into the earth to dig a pit for her home, Na-maka-o-kaha`i,
goddess of water and the sea, would flood the pits.
Pele moved down the chain of islands in order of their
geological formation, eventually landing on the Big
Island's Mauna Loa, which is considered the tallest
mountain on Earth when measured from its base at the
bottom of the ocean (Betty Fullard-Leo, 1999;
http://www.coffeetimes.com/pele.htm). |
Historical background
The distinctive northwest-southeast alignment of
the Hawaiian chain was known to the early Hawaiians.
Their legends clearly reveal that they recognized
that the islands are progressively younger from the
northwest to the southeast.
The first geologic study of the Hawaiian Islands
(1840-1841) was directed by James Dwight Dana who
deduced that the islands young to the southeast from
the differences in their degree of erosion. He also
suggested that some other island chains in the Pacific
showed a similar general decrease in age from northwest
to southeast.
The Hawaiian chain apparently consists of two strands
of volcanoes located along distinct but parallel curving
pathways. Multiple volcanoes line up to form each
strand. Dana coined the terms Loa and Kea series for
two of the prominent trends. The Kea trend includes
the volcanoes of Kilauea, Mauna Kea, Kohala, East
Maui (Haleakala) and West Maui. The Loa trend includes
Lo`ihi, Mauna Loa, Hualalai, Mahukona (a submerged
volcano), Kaho`olawe, Lana`i, and West Moloka`i (Figure
1). A pair of volcano trends may exist all the way
along the Hawaiian and Emperor chains, though this
is less clear amongst the older islands and seamounts
[Clague & Dalrymple, 1987].
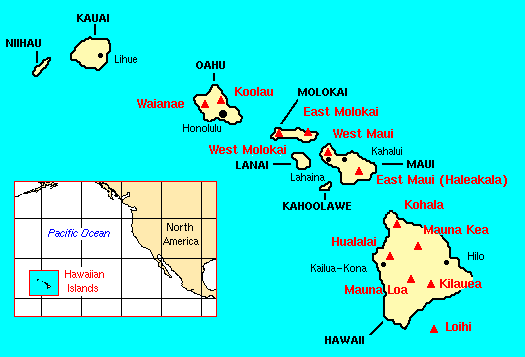
Figure 1: The Hawaiian islands and principle
volcanoes.
|
The alignment of the Hawaiian Islands, Dana proposed,
reflected localized volcanic activity along segments
of a major fissure zone on the ocean floor. Dana's
“great fissure” origin for the islands
served as a working hypothesis for subsequent studies
until the mid-20th century.
In 1963, Tuzo Wilson pointed out that the time-progressive
volcanism along the Hawaiian chain could be explained
by the lithosphere moving across a stationary hot
spot in the mantle [Wilson, 1963]. This suggestion
gave rise, a decade later, to the theory of deep mantle
plumes when Morgan [1971;
1972] proposed that a) this hot spot was continually
supplied by a plume from the deep mantle, and b) there
are approximately 20 such plumes in the mantle. Fixity
relative to one-another, time-progressive volcanic
tracks, and a high rate of volcanism were considered
to be the primary characteristics of volcanic regions
fuelled by deep-mantle plumes.
The Hawaiian - Emperor system appears superficially
to fit the fixed deep mantle plume hypothesis well,
and indeed, the hypothesis was inspired by it. The
most compelling and widely quoted evidence today is
still geometric considerations such as fixity, perceived
parallelism with other volcanic chains and the regular
time progression of volcanism, along with the high
melt productivity. Lnear time-progression of volcanism
and high magmatic productivity can be explained by
other mechanisms such as propagating cracks and high
mantle fertility. Nevertheless, for about 20 years
there has been no serious challenge to the fluid-dynamic,
deep thermal mantle plume hypothesis for the origin
of the Emperor and Hawaiian islands and seamounts.
There are, however, a substantial number of aspects
of the chains that are not predicted by the plume
hypothesis and fit it poorly. These must give clues
to alternative genesis models that would be interesting
to test, and might satisfy the observations better.
This review summarizes those, and other, aspects of
the Emperor and Hawaiian chains.
|
Aspects
of the Emperor and Hawaiian chains unpredicted by the
plume hypothesis
1. The “bend”
did not result from a change in direction of Pacific
plate motion: The Emperor and Hawaiian chains differ
in trend by about 60°. The two trends intersect
at about 30°N, near the Mendocino fracture zone
(Figures 3 & 4). It is often assumed that this resulted
from a change in direction of motion of the Pacific
plate at ~ 50 Ma. However, such a change in plate direction
did not occur, as is shown by magnetic lineations, fracture
zone orientations and plate motion reconstructions [e.g.,
Raymond et al., 2000]. This “nonevent”
[Norton, 1995] is often attributed to the collision
of India with Asia or to changes in circumpacific geometry
[Gordon et al., 1978]. In any case, reorganisation
of global plate movements resulting from continental
collision is not expected to be a geologically instantaneous
change from one stable state to another as would be
required if the bend is to be explained in this way
[Duncan & Richards, 1991; Lithgow-Bertelloni
& Richards, 1995; Richards & Lithgow-Bertelloni,
1996]. Rapid and large changes in plate motion are not
expected, given current understanding of plate forces.
Convective forces can change instantaneously, but plate
driving forces cannot.
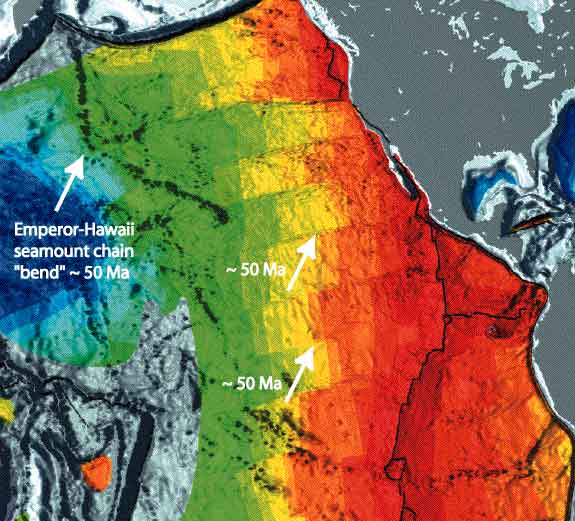
Figure 3: Map of the Pacific ocean showing
sea floor age as determined from magnetic lineations.
It can be seen clearly from the continuity of
fracture zones that no change in the direction
of motion of the Pacific plate occurred at the
time of the bend at ~ 50 Ma.
|
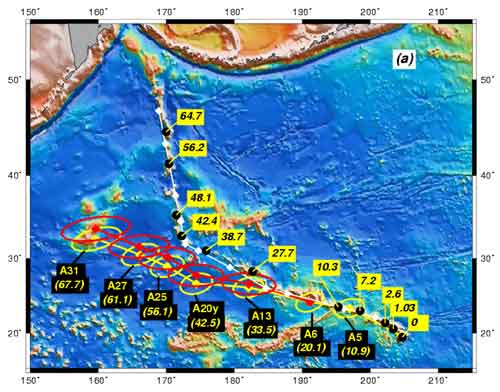
Figure 4: Locations of seamounts and volcanoes
of various ages on the Emperor and Hawaiian
chains, along with their their theoretical positions,
calculated from reconstructions of global plate
motions, assuming a fixed locaation of the “hotspot”
relative to a fixed Indo-Atlantic hotspot reference
frame [from Raymond et al., 2000].
|
In addition to the change in trend,
the locus of volcanism moved south by ~ 800 km relative
to the geomagnetic and biofacies reference frames while
the Emperor Seamount chain formed. This conclusion is
supported by data from bioclastic sediment on Emperor
seamounts, which corresponds to higher latitudes than
present-day Hawaii [Butt, 1980; McKenzie
et al., 1980]. Prior to the bend, the hotspot migrated
at ~ 7 cm/year relative to the Pacific sea floor. The
rate of propagation changed at the time of the bend
to ~ 9 cm/year.
The Hawaiian and Emperor chains appear
thus to be separate phenomena in some sense. Their orientations
might be controlled by the direction of regional stress,
the fabric of the seafloor, or stresses caused by previously
erupted volcanoes [Hieronymus & Bercovici,
1999]. Fluctuations also occur in the directions and
volumes of individual chains and volcanoes [Clague
& Dalrymple, 1987].
2. The Emperor
chain began near a ridge: 86Sr/ 87Sr
is uniform along most of the volcanic chain, but
decreases to MORB-like values on approaching the
~ 80 Ma Detroit seamount, the oldest (and largest)
of the dated Emperor seamounts. Pacific plate
palinspastic reconstructions [ Engebretson
et al., 1985; Smith, 2003] (see
Pacific page),
and the elastic thickness of the lithosphere beneath
the northern Emperors [ Watts, 1978],
are consistent with the Detroit seamount having
been erupted on or very close to a spreading ridge. |
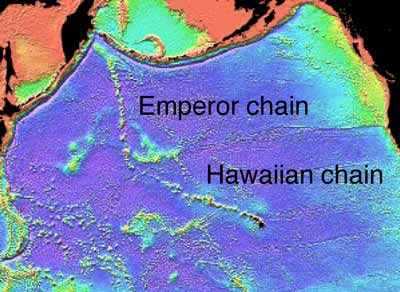
Figure 5: Pacific bathymetry
clearly shows the Emperor and Hawaiian volcanic
chains. |
This is a coincidence
in the plume hypothesis, and is not unique to
Hawaii. Many other hotspots are on ridges or their
tracks started on ridges. The “primary hotspots”
of Courtillot
et al. [2003] are mainly on ridges, plate
boundaries, triple junctions, or fracture zones
near plate boundaries. |
3. There is no Emperor/Hawaiian
“plume head”: Flood-basalt provinces
and oceanic plateaus are commonly thought to represent
the initial stages of plume volcanism [Campbell
& Griffiths, 1990; Campbell & Griffiths,
1993]. If the Hawaiian-Emperor chain began with such
extensive volcanism, the plateau so produced is no longer
present on the Pacific plate. Oceanic plateaus are not
thought to be subductable, and thus an oceanic “plume
head” for the Emperor chain would presumably have
been scraped off, accreted or obducted onto the Aleutian/Kurile/Kamchatka
arc. There is no evidence for such material associated
with the Emperor chain although other accreted plateaus,
e.g. Wrangellia in the Pacific NE, have been identified.
Some alternative hypotheses, e.g., propagating cracks,
do not require a large igneous province at the beginning
of the chain. Many other hotspot tracks also lack a
“plume head” (e.g., the Easter hotspot),
and many oceanic plateaus and large igneous provinces
on land lack an obvious “tail”, or “hotspot
track” (e.g., the Siberian
Traps). |
4. The magmatic rate is highly variable:
The eruption rate along the Emperor Seamount chain
averaged ~ 0.01 km3/yr [Bargar &
Jackson, 1974] (Figure 6). The eruption rate
was very low, almost zero, for the initial ~ 5 Myr
of the Hawaiian chain, but the average along the Hawaiian
chain has been 0.017 km3/yr. Over the last
~ 6 Myr it has been higher than ever before, or 0.095
km3/yr, but the average rate for the last
1 Myr is even higher, or 0.21 km3/yr [Robinson
& Eakins, 2006]. These rates may be compared
with the current Pu'u 'O'o eruption rates of 0.113
km3/yr and a value also attributed to the
shield stage of Hawaiian volcanism. The average magmatic
production rate at a mid-ocean ridge spreading segment
is ~ 0.02 km3/yr.
The eruption rate may correlate with
the propagation rate of the melt locus, as this has
approximately doubled over the last 2 Myr [Shaw
et al., 1980; Clague & Dalrymple,
1987]. The southeast end of the Hawaiian chain is
surrounded by a broad area of seafloor containing
smaller-volume contemporaneous volcanism. These large
variations in magmatic rate illustrate that the current
magmatic rate is highly anomalous and should not be
considered to be "typical" hot spot or plume
behaviour. They are not currently explained.
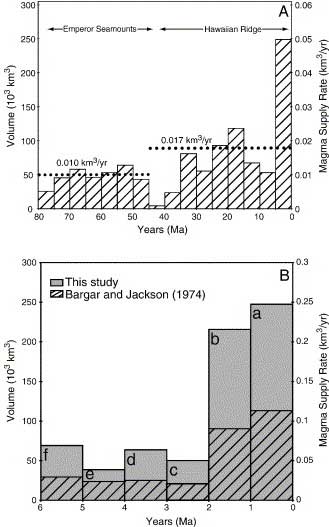
Figure 6: Calculated volumes
and magma supply rates. (A) Histogram along
the Emperor Seamounts and Hawaiian Ridge at
5-my intervals (from Bargar and Jackson, 1974).
Dotted lines mark the long-term average magma
supply rates. (B) Histogram of the Hawaiian
Islands at 1-my intervals. Intervals roughly
correspond to individual island complexes
and volcanoes: (a) Hawaii Island, (b) Maui
Nui, (c) Koolau, (d) Waianae, (e) Niihau and
Kaula, (f) Kauai. Gray bars show volumes and
magma supply rates for this study; diagonal
lines show results from Bargar and Jackson
(1974). Both studies show the same trend of
increasing magma supply rates towards the
present (from Robinson
& Eakins, 2006).
|
No quantitative model has explained the flux rate
and its variation at Hawaii and along the Hawaiian
and Emperor chains. No thermal model has explained
how high flux rates can occur beneath thick plates,
where the top of the productive part of the melting
column is missing. The plume-head/plume-tail hypothesis
predicts a large initial rate that declines subsequently,
the opposite of that observed along the Hawaiian chain.
|
5. There is no heatflow anomaly: Lithosphere
underlain by a thermal plume is expected to be thinner
and to have higher heat flow than the average for
lithosphere of the same age elsewhere. Heatflow across
the Hawaiian swell, however, shows no significant
anomaly [von Herzen et al., 1989; Stein
& Stein, 1992,1993; McNutt,
2000] (see Heatflow page).
The bathymetric swell surrounding the southernmost
part of the Hawaiian chain cannot therefore be explained
as a thermal effect, and in the plume model must be
attributed to dynamic or compositional effects with
no discernible thermal effect shallower than the base
of the ~ 80-km-thick lithosphere [Liu & Chase,
1989; Sleep, 1994] (Figure 7). Anomalous
heat flow and thermal rejuvenation (i.e., thinning
of the plate) are predicted by the plume hypothesis
[Crough, 1983] but not by some alternative
hypotheses e.g., propagating cracks.
Figure 7: Intermediate wavelength
geoid anomalies near the Hawaiian chain. |
6. Mantle temperature is elevated by up to ~
200°C: Petrology can be used to infer the
temperature where surface-erupted melts were last
in equilibrium with the mantle source, and Tp, the
mantle potential temperature. (see Temperature
page) The results for Hawaii are variable, and
whether or not a high temperature anomaly is inferred
depends on what “average” mantle temperature
is considered to be. “Average” mantle
temperature is mostly studied at mid-ocean ridges
(MORs), since this is where magma of mantle origin
is mostly erupted. The temperatures required for plumes
to rise are debated. Peridotic plumes from the core-mantle
boundary would require temperature anomalies of the
order of 600 K [Watson & McKenzie, 1991;
Cordery et al., 1997]. Temperature anomalies
of ~ 250 K have been suggested for crust-contaminated
plumes rising from the transition zone [e.g., Courtney
& White, 1986], but it is debatable whether
such a phenomenon is physically possible as the base
of the upper mantle is not thought to be a thermal
boundary layer.
Combinations of geophysical and geochemical arguments
(e.g., “garnet signature” in rare-Earth
elements, olivine-liquid
geothermometers have been used to infer temperatures
beneath Hawaii of 150 - 200 K higher than MORs. Also,
study of the MgO contents of picritic glass found
on the Puna ridge (the submarine part of Kilauea's
east rift zone) indicate that if they represent primary
melts from a lherzolite source in the presence of
H2O and CO2, their minimum potential
temperature of generation was ~ 1420°C [Gudfinnsson
& Presnall,
2002]. This suggests formation at a potential
temperature 140-160°C hotter than at MORs if an
average Tp of 1260-1280°C is assumed [e.g., McKenzie
& Bickle, 1988; Presnall et al.,
2002].
This conclusion is dependent on the “average”
mantle Tp assumed. Study of basalt petrogenesis by
high-pressure experimental methods, in which both
the progressive melting behaviour of peridotite and
(C-H-O) is investigated, has been applied to both
Hawaii and MORs. The results, coupled with data from
olivine phenocrysts in Hawaiian picrites, suggest
temperatures of formation of ~ 1325°C for both
Hawaiian and MOR magmas, and a temperature difference
no more than ~ 20 K [Green et al., 2001]
(see Mantle temperature
page).
Hawaii is the only oceanic island where picrite glass
has been found. It is not abundant, however, and is
only known from a few grains of turbidite sand [Clague
et al., 1991; Clague et al., 1995].
The presence of picrite glass suggests that Hawaii
is hotter than localities that lack picrite glass.
However, Hawaii is better sampled than almost any
other oceanic hotspot on Earth, and given the extreme
rarity (one location) of this lithology there, it
is not safe to conclude from this that all other chains
are cooler.
The bottom line today is that the majority of studies
suggest that there is a temperature anomaly beneath
Hawaii today, compared with MORs, but it is less than
200 K, which is somewhat less than that generally
assumed to be required in the coolest of plumes. The
temperature variations in the mantle expected from
normal plate tectonic processes are ± 180ÚC
[Kaula, 1983].
|
Lava at Kilauea. Photo by
Hetu Sheth |
7. The
melt originates from the shallow asthenosphere:
The petrology of Hawaiian lavas requires that
much of the melt was last in equilibrium with
its mantle host in the shallower part of the
garnet stability field. It rules out an origin
deep in the garnet stability field, meaning
that the melt probably comes from ~ 80 –
120 km depth, or near the base of the lithosphere.
(e.g., T.
Sisson, unpublished results) The greater
the depth of melting and the larger the melt
drainage area to Hawaiian volcanoes, the larger
the potential melt volume, for whatever mechanism
is proposed. |
An isolated
one-dimensional volcanic feature such as Hawaii
can drain a volume larger than a two-dimensional
structure such as a ridge, which must share
its magma sources with adjacent ridge segments. |
|
8. OIB geochemistry is ambivalent regarding
depth of origin: Ocean-island basalt (OIB) geochemistry
by itself does not require a mantle plume, or a deep
or hot source. The ~ 80 – 120 km depth of melting
indicates that the immediate source of the “enriched”
signature of OIB is the shallow mantle. What is disputed
is the ultimate origin of material - whether it is
the deepest mantle or the mantle wedge, the asthenosphere
or a shallower layer which collects subduction zone
products. Hawaiian lavas exhibit both enriched and
depleted signatures showing chemical similarities
to continental and marine sediments, ocean crust and
ridge basalts. This collection of signatures defines
OIB geochemistry, two of the main characteristics
of which are a large statistical variance in isotopic
ratios, and rapid lateral and temporal differences.
OIB geochemistry has been attributed to the incorporation
of subducted oceanic crust or sediments into the mantle
source [Hofmann & White, 1982]. In the
deep-plume model, these materials are carried to the
core-mantle boundary, through the homogeneous depleted
upper mantle, and then they ascend all the way back
up in the core of the plume. This mechanism is inconsistent
with the observation that OIB is widespread throughout
the Pacific, occurring on thousands of seamounts where
plumes are neither expected nor reasonable to postulate.
The geochemistry of lavas from the Hawaiian and Emperor
chains changes:
-
geographically, where the chain
crosses fracture zones, e.g., the Mendocino, Murray
and Molakai fracture zones [Basu & Faggart,
1996], mainly by increase in the variance,
-
temporally, e.g., Mukhopadhyay
et al. [2003] report variations in 3He/4He
of up to 8 Ra during a single century in Kauai volcano,
that correlate with variations in radiogenic isotope
ratios. This suggests rapid changes in composition
or contamination of the melt supplying the magma
reservoir, and
-
spatially, from volcano to volcano,
such that different volcanoes do not appear to be
fed by the same magma source.
“End-member” and principal-component
interpretations require at least four different source
components to explain the geochemistry of Hawaiian
lavas. This result, along with the variability described
above, suggests a spatially distributed, compositionally
inhomogenous and temporally variable source that is
also sensitive to shallow lithospheric features. A
chemically heterogeneous shallow mantle, due to recycling
[Meibom
& Anderson,
2003] or a zoned plume stem have been proposed.
The maximum 3He/4He observed
at Hawaii is 35 Ra in samples from Loihi [Graham,
2002]. Such high maximum ratios are often cited as
unambiguous evidence for lower-mantle affinity and
a deep-mantle plume. This model is disputed (see Helium
Fundamentals page).
|
9. Seismology
has not detected a plume: The most promising seismic
methods for detecting plume-like structures are:
-
teleseismic tomography, which
can image the three-dimensional wave-speed in the
upper few hundred kilometers,
-
receiver functions (waves converted
at upper mantle discontinuities), which can reveal
topography on the 410- and 650-km discontinuities,
and the thickness of the transition zone, and
-
A hot column of mantle should reveal
low wave speeds wherever earthquake waves cross it,
and should depress the 410 km discontinuity and elevate
the 650 km discontinuity, because of the effect of temperature
on phase boundaries. A large active upwelling would
spread out below the lithosphere, and be dragged to
the NW. Actual tomography shows extension of the low-velocity
zone to the SE, toward the East Pacific Rise.
Early teleseismic tomography on the
big island of Hawaii revealed little structure in the
upper ~ 80 km, and poorly resolved lower velocities
below this [Ellsworth, 1977]. An experiment
using a network ~ 600 km long, involving digital seismic
stations on several of the islands detected a low-wave-speed
anomaly beneath the islands of Maui and Molokai, 250
km NW of the big island, but no low-wave-speed anomaly
beneath the big island down to the maximum depth of
good resolution there at ~ 150 km [Wolfe et al.,
2002] (Figure 8).
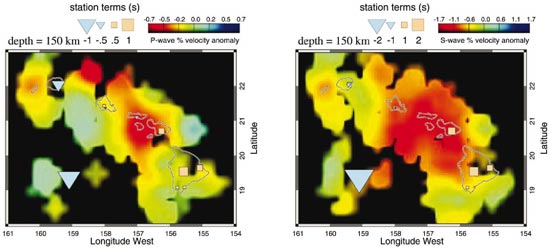
Figure 8: Seismic anomalies beneath the
Hawaiian island archipelego at 150 km depth
[from Wolfe et al., 2002].
|
In order to probe the upper mantle
in detail throughout much of its thickness, a ~ 600-km-wide
network including many ocean-bottom seismometers is
required. Such an experiment is currently underway (see
http://obsmac1.whoi.edu/~bobd/plume.html).
Tomography in the central Pacific can
only provide relatively good resolution of upper-mantle
wave speeds if surface waves and multiple ScS
waves are used, and can resolve structures on a spatial
scale of a few hundred kilometers. Cross sections through
the best available model of this sort - S20RTS [Ritsema
et al., 1999] are shown in Figure 9.
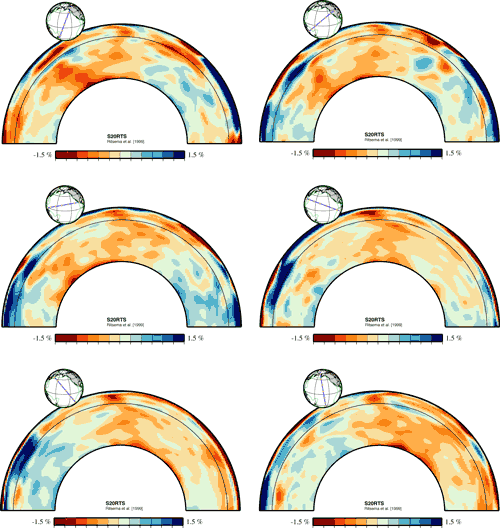
Figure 9: Six cross sections passing through
the big island of Hawaii and the whole-mantle
tomography model of Ritsema et al. [1999]. The
big island is exactly in the centre of the cross
sections.
|
A strong low-wave-speed anomaly lies
to the NW of the big island of Hawaii, which might be
associated with that detected by Wolfe et al.
[2002]. There is apparent continuity of low-wave-speed
material between the surface in the region of Hawaii
and the core-mantle boundary beneath a large swath of
the Earth ranging from the New Hebrides and Samoa, throughout
the south Pacific and north along the East Pacific Rise,
depending on the line of cross section selected. These
connections involve various anomaly contortions and
tilts. From the standpoint of the whole-mantle plume
model, the problem lies in choosing which of many candidate
anomalies to advocate, rather than explaining why one
is not detected, as is the case for other hotspots
such as Iceland and Tristan
da Cunha.
A magnitude 6.2 earthquake occurred
on the big island in 1973, and generated shear (S)
waves that travelled directly downwards, reflected from
the boundary of the core and, upon their return, registered
on a seismometer on the big island (ScS waves).
Six multiple echoes were well recorded. The times of
these waves indicate that the average S-wave
speed of the mantle beneath the Hawaii region [Katzman
et al., 1988] is somewhat higher than the average
beneath the southwestern Pacific Ocean [Best et
al., 1975], and that the propagation efficiency
is high, contrary to expectations for regions of high
temperature or partial melting. (see Julian
& Foulger, 2003).
Transition zone thickness beneath the
Hawaii region is estimated to be 229 km [Gu
& Dziewonski,
2001]. This is ~ 13 km thinner than the global average
of 242 km, but not significantly thinner than the transition
zone throughout much of the central Pacific and other
oceans (Figure 10) (see also Transition
zone page).
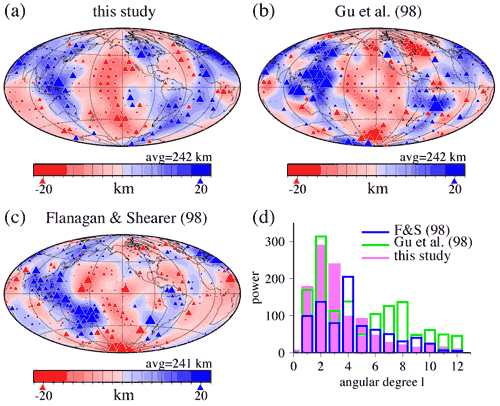
Figure 10: Thickness of the transition
zone. Residuals are interpolated using a spherical
harmonic expansion up to degree 12. The long-wavelength
features are fairly consistent among these maps,
which are dominated by low-degree harmonics
[from Gu
& Dziewonski, 2001].
|
In summary, the results of seismic
studies of the mantle beneath the Hawaiian region to
date suggest that:
- the Pacific as a whole, especially the south Pacific,
is a region of anomalously low wave speeds on a
global scale,
- low wave-speed anomalies at the surface in the
Hawaii region are continuous down to the core-mantle
boundary along numerous paths that embrace almost
the entire south Pacific,
- ironically, the average wave speed immediately
beneath the Hawaiian island region itself is not
unusually low, and
- the transition zone is somewhat thinner than the
global average but typical of the central Pacific
as a whole.
|
Tomography
& Geochemistry
Variations in the geochemistry
of Hawaiian lavas have been interpreted in the
context of a zoned plume model. This approach
predicts a plume less than 50 kilometers in diameter
[DePaolo et al., 2001] (Figure 11). Such
a plume would be undetectable seismically, except
in the upper 50-100 km, where a low-wave-speed
anomaly is not detected in tomographic studies.
If such a plume were downward-continuous with
a similar diameter, it would be too small to be
detected by teleseismic tomography using a broad
ocean-bottom seismic network.
(http://obsmac1.whoi.edu/~bobd/plume.html).
Figure 11: Geochemical "plume maps"
(from DePaolo et al., 2001) |
|
|
Other
possible constraints or coincidences
-
The Hawaiian chain is bounded
by a large gravitational moat and arch structure
that is most strongly evident in bathymetry around
its southeastern end (Figure 7).
-
Some Hawaiian basalts and xenoliths
have extreme 3He/4He ratios
compared with the MORB mean. In common with other
“hotspots” these also exhibit high variance
and have lower 3He abundances than MORB
glasses.
-
The volcanoes of the Hawaiian
chain, and possibly also the Emperors, feature long,
nested, lateral rift zones which are rarely seen
on other oceanic islands (see Samoa
page).
-
Hawaiian volcanoes are distributed
along the paired Loa and Kea trends that comprise
arrays of sinusoidal volcano loci. These trends
appear to be controlled by the loads of the volcanic
edifices [Hieronymus & Bercovici, 1999].
-
The volcanic constructs (groups
of volcanoes) along the chains are mostly oriented
at a high angle to the overall trend of the volcanic
chain. The orientations of the rift systems are
mostly oblique to structure on the underlying seafloor
such as ridge segments and transform faults.
-
Hawaii is about as far away
from other active volcanic chains, hotspots, plate
boundaries of all types and continents as it is
possible to be, very close to the center of the
colossal Pacific plate.
-
The oldest end of the Emperor
chain is subducting almost exactly into the cusp
of the Aleutian/Kurile trench – the “NW
Pacific pocket”. The cause and effect of this
are debated.
-
The great bend occurs close
to where the chain crosses the Mendocino fracture
zone.
|
Summary
What, then, is the evidence in support
of a deep mantle plume? The Hawaiian hotspot has a very
regular time-progressive track. Clearly, during the
last ~ 50 Myr the melt anomaly has remained approximately
stationary with respect to the North American plate,
beneath which the Pacific plate is subducting at the
Aleutian trench. (Since the Pacific plate is moving
much faster than any other plate on Earth, the Hawaiian
hotspot is essentially fixed with respect to every other
plate also.) The magmatic rate is currently very high,
and is characterised by OIB geochemistry. This includes
high maximum 3He/4He isotope ratios,
commonly considered to be a “smoking gun”
for a plume from the lower mantle.
Alternative mechanisms have been proposed
for all these observations (see also Cracks
& Stress page). Other Pacific volcanic chains
roughly parallel to the Hawaiian chain may (e.g., the
eastern end of the Louisville chain) or may not (e.g.,
the Line Islands) be time-progressive. High rates of
melt production have been explained by fertile mantle
composition, perhaps resulting from remelting subducted
oceanic crust, and this would also explain OIB geochemistry.
Shallow models for high 3He/4He
isotope ratios, e.g., release of old helium stored for
100s of Myr in subducted, lower-crustal, olivine-rich
cumulates, have also been proposed and not challenged
to date [e.g., Anderson,
1998a; Anderson,
1998b; Meibom et al., 2003] (see Helium
Fundamentals page). However, a persuasive, alternative
model for the Emperor and Hawaiian volcanic chains remains
to be fully quantified.
Any satisfactory theory for Hawaiian
volcanism must explain (or rationalize) the:
-
change in migration direction
of the melting locus at the bend,
-
association of the great bend
with the Mendocino fracture zone,
-
change in migration rate at
the bend,
-
apparent commencement of the
volcanic chain near a ridge,
-
absence of a “plume head”,
-
large variations in magmatic
production, and a current magmatic rate about 3
times greater than the next most productive hotspots,
-
absence of a significant heat
flow anomaly,
-
absence of lithospheric thinning,
-
absence of a strong high-temperature
signal in the erupted basalts,
-
production of very large volumes
of magma even though the depth to the top of the
melting column is exceptionally large compared with
MORs,
-
spatial and temporal variation
in the composition of erupted lavas on a variety
of scales,
-
remote location of Hawaii, near
the center of a very large plate,
-
location of the oldest end of
the chain with respect to the “Pacific pocket”,
-
unique rift zones,
-
paired Loa and Kea trends,
-
seismic whole-mantle mantle
structure that is apparently normal compared with
the Pacific ocean elsewhere, and
-
occurrence of a bathymetric swell
(a moat and “arch”) along the eastern
two-thirds of the Hawaiian chain and wrapping around
its southeastern end, with alkalic basaltic volcanism
occurring at some places along it.
In conclusion, Hawaii is not fully
explained by any current hypothesis. It is impressive
that a region of the Earth so extensively studied for
so many years, by so many Earth scientists with so many
techniques could remain so intransigent to full understanding.
Many of the numerous features that are not yet fully
understood, and the parameters of alternative hypotheses,
are not currently being studied, but they offer exciting
research opportunities. |
Acknowledgments:
We gratefully acknowledge the input and help of Jim
Natland, Dean Presnall, Bruce Julian, Warren Hamilton
and Seth Stein in drafting this web page. This acknowledgement
does not necessarily imply agreement with its contents.
We also thank Barry Eakins, Joanne Stock, Jim Natland
and Jeroen Ritsema for supplying figures. |
News
& Discussion
"Fixed"
Hawaiian hotspot not fixed |
-
-
-
Bargar, K.E., and E.D. Jackson,
Calculated volumes of individual shield volcanoes
along the Hawaiian-Emperor chain, U.S. Geological
Survey Journal of Research, 2,
545-550, 1974.
-
Basu, A.R., and Faggart, Temporal
Variation in the Hawaiian mantle plume: The Lanai
anomaly, the Molokai fracture zone and a seawater-altered
lithospheric component, in Hawaiian volcanism,
in Earth Processes: Reading the Isotopic Code,
edited by A. Basu, and S. Hart, pp. 149-159, 1996.
-
Best, W.J., L.R. Johnson, and
T.V. McEvilly, ScS and the mantle beneath
Hawaii, EOS Trans. AGU, 1147, 1975.
-
Butt, A., Biostratigraphic and
paleo-environment analyses of the sediments at the
Emperor Seamounts, DSDP Leg 55, Northwestern Pacific:
Cenozoic foraminifers, in Initial Reports, DSDP,
edited by E.D. Jackson, I. Koizumi, and others,
pp. 289-325, U.S. Government Printing Office, Washington,
1980.
-
Campbell, I.H., and R.W. Griffiths,
Implications of mantle plume structure for the evolution
of flood basalts, Earth planet. Sci. Lett.,
99, 79-93, 1990.
-
Campbell, I.H., and R.W. Griffiths,
The evolution of the mantle's chemical structure,
Lithos, 30, 389-399, 1993.
-
Clague, D.A., and G.B. Dalrymple,
The Hawaiian-Emperor volcanic chain, in Volcanism
in Hawaii, edited by R.W. Decker, T.L. Wright,
and P.H. Stauffer, pp. 5-54, U.S. Government Printing
Office, 1987.
-
Clague, D.A., J.G. Moore, J.E.
Dixon, and W.B. Friesen, Petrology of submarine
lavas from Kilauea's Puna ridge, Hawaii, J.
Pet., 36, 299-349, 1995.
-
Clague, D.A., W.S. Weber, and
J.E. Dixon, Picritic glasses from Hawaii, Nature,
353, 553-556, 1991.
-
Cordery, M.J., G.F. Davies, and
I.H. Campbell, Genesis of flood basalts from eclogite-bearing
mantle plumes, J. geophys. Res., 102,
20,179-20,197, 1997.
-
Courtillot, V., A. Davaillie,
J. Besse, and J. Stock, Three distinct types of
hotspots in the Earth's mantle, Earth planet.
Sci. Lett., 205, 295-308,
2003.
-
Courtney, R.C., and R.S. White,
Anomalous heat flow and geoid across the Cape Verde
Rise: evidence fro dynamic support from a thermal
plume in the mantle, Geophys. J.R. Astron. Soc.,
87, 815-867, and microfiche GJ
87/1, 1986.
-
Crough, S.T., Hotspot swells,
Ann. Rev. Earth Planet. Sci., 11,
165-193, 1983.
-
DePaolo, D.J., J.G. Bruce, A.
Dodson, D.L. Shuster, and B.M. Kennedy, Isotopic
evolution of Mauna Loa and the chemical structure
of the Hawaiian plume, Geochemistry, Geophysics,
Geosystems, 2, 2000GC000139,
2001.
-
Duncan, R.A., and M.A. Richards,
Hotspots, mantle plumes, flood basalts, and true
polar wander, Rev. Geophys., 29,
31-50, 1991.
-
Ellsworth, W.L., Three-dimensional
structure of the crust and mantle beneath the island
of Hawaii, Ph.D. thesis, Massachusetts Institute
of Technology, 1977.
-
Engebretson, D.C., A. Cox, and
R.G. Gordon, Relative motions between oceanic and
continental plates in the Pacific basin, in Geol.
Soc. Am. Spec. Pap., pp. 1-59, 1985.
-
Gordon, R.G., A.A. Cox, and C.E.
Harter, Absolute motion of an individual plate estimated
from its ridge and trench boundaries, Nature,
274, 752-755, 1978.
-
Graham, D.W., Noble gas isotope
geochemistry of mid-ocean ridge and ocean island
basalts: Characterization of mantle source reservoirs,
Noble gases in Geochem. Cosmochem., Rev. Min.
Geochem., 47, 247-317, 2002.
-
Green, D.H., T.J. Falloon, S.M.
Eggins, and G.M. Yaxley, Primary magmas and mantle
temperatures, Eur. J. Min, 13,
437-451, 2001.
-
-
-
Hieronymus, C.F., and D. Bercovici,
Discrete alternating hotspot islands formed by interaction
of magma transport and lithospheric flexure, Nature,
397, 604-607, 1999.
-
Hofmann, A.W., and W.M. White,
Mantle plumes from ancient oceanic crust, Earth
planet. Sci. Lett., 57, 421-436,
1982.
-
-
Katzman, R., L. Zhao, and T.H.
Jordan, High-resolution, two-dimensional vertical
tomography of the central Pacific mantle using ScS
reverberations and frequency-dependent travel times,
J. geophys. Res., 103,
17,933-17,971, 1988.
-
Kaula, W.M., Minimal upper mantle
temperature variations consistent with observed
heat flow and plate velocities, J. geophys.
Res., 88, 10,323-10,332, 1983.
-
Lithgow-Bertelloni, C., and M.A.
Richards, Cenozoic plate driving forces, Geophys.
Res. Lett., 22, 1317-1320,
1995.
-
Liu, M., and C.G. Chase, Evolution
of midplate hotspot swells - numerical solutions,
J. geophys. Res., 94,
5571-5584, 1989.
-
McKenzie, D., and J. Bickle,
The volume and composition of melt generated by
extension of the lithosphere, J. Pet.,
29, 625-679, 1988.
-
McKenzie, J.A., D. Bernoulli,
and S.O. Schlanger, Shallow-water carbonate sediments
from the Emperor Seamounts: Their diagenesis and
paleogragraphic significance, in Initial Reports,
DSDP, edited by E.D. Jackson, I. Koizumi, and
others, pp. 415-455, U.S. Government Printing Office,
Washington, 1980.
-
-
-
Meibom,
A., D.L. Anderson, N.H. Sleep, R. Frei, C.P. Chamberlain,
M.T. Hren, and J.L. Wooden, Are high 3He/4He
ratios in oceanic basalts an indicator of deep-mantle
plume components?, Earth planet. Sci. Lett.,
in press, 2003.
-
Morgan, W.J., Convection plumes
in the lower mantle, Nature, 230,
42-43, 1971.
-
Morgan, W.J., Deep mantle convection
plumes and plate motions, Bull. Am. Assoc. Pet.
Geol., 56, 203-213, 1972.
-
Mukhopadhyay, S., J.C. Lassiter,
K.A. Farley, and S.W. Bogue, Geochemistry of Kauai
shield-stage lavas: Implications for the chemical
evolution of the Hawaiian plume, Geochemistry,
Geophysics, Geosystems, 4,
10.1029/2002GC000342, 2003.
-
Norton, I.O., Plate motions in
the North Pacific; the 43 Ma nonevent, Tectonics,
14, 1080-1094, 1995.
-
Presnall, D.C., G.H. Gudfinnsson,
and M.J. Walter, Generation of mid-ocean ridge basalts
at pressures from 1 to 7 GPa, Geochim. Cosmochim.
Acta, 66, 2073-2090, 2002.
-
Raymond, C.A., J.M. Stock, and
S.C. Cande, Fast Paleogene motion of the Pacific
hotspots from revised global plate circuit constraints,
in History and Dynamics of Plate Motions,
edited by M.A. Richards, R.G. Gordon, and R.D. van
der Hilst, pp. 359-375, 2000.
-
Richards, M.A., and C. Lithgow-Bertelloni,
Plate motion changes, the Hawaiian-Emperor bend,
and the apparent success of dynamical models, Earth
planet. Sci. Lett., 137, 19-28,
1996.
-
Ritsema, J., H.J. van Heijst,
and J.H. Woodhouse, Complex shear wave velocity
structure imaged beneath Africa and Iceland, Science,
286, 1925-1928, 1999.
-
-
Shaw, H.R., E.D. Jackson, and
K.E. Bargar, Volcanic periodicity along the Hawaiian-Emperor
chain, Am. J. Sci., 280-A,
667-708, 1980.
-
Sleep, N.H., Lithospheric thinning
by midplate mantle plumes and the thermal history
of hot plume material ponded at sublithospheric
depths, J. geophys. Res., 99,
9327-9343, 1994.
-
Smith, A.D., A re-appraisal of
stress field and convective roll models for the
origin and distribution of Cretaceous to Recent
intraplate volcanism in the Pacific basin, Int.
Geol. Rev., 45, in press,
2003.
-
Stein, C.A., and S. Stein, A
model for the global variation in oceanic depth
and heat-flow with lithospheric age, Nature,
359, 123-129, 1992.
-
Stein, C.A. and Stein, S., Constraints
on Pacific midplate swells from global depth-age
and heat flow-age models, in The Mesozoic Pacific:
Geology, Tectonics, and Volcanism, Geophysical
Monograph. American Geophysical Union, Washington,
D.C., pp. 53-76, 1993.
-
von Herzen, R.P., M.J. Cordery,
R.S. Detrick, and C. Fang, Heat-flow and the thermal
origin of hot spot swells - the Hawaiian swell revisited,
J. geophys. Res., 94, 13,783-13,799,
1989.
-
Watson, S., and D. McKenzie,
Melt generation by plumes: a study of Hawaiian volcanism,
J. Pet., 32, 501-537,
1991.
-
Watts, A.B., An analysis of isostasy
in the world's oceans; 1, Hawaiian-Emperor seamount
chain, J. geophys. Res., 83,
5989-6004, 1978.
-
Wilson, J.T., A possible origin
of the Hawaiian Islands, Can. J. Phys.,
41, 863-870, 1963.
-
Wolfe, C.J., S.C. Solomon, P.G.
Silver, J.C. VanDecar, and R.M. Russo, Inversion
of body-wave delay times for mantle structure beneath
the Hawaiian islands: results from the PELENET experiment,
Earth planet. Sci. Lett., 198,
129-145, 2002.
|
last updated
11th March, 2006 |
|
|